Polyelectrolyte Multilayer Films and Membrane Functionalization
Merlin L. Bruening, Maneesha Adusumilli
Department of Chemistry, Michigan State University East Lansing, MI 48824
Material Matters Volume 6 Number 3
Introduction
Among the many methods for preparing thin films, layer-by-layer (LbL) deposition of complementary polymers has emerged as an especially versatile technique for controlling film thickness and functionality. Figure 1 shows the most common form of the LbL method, which is the alternating adsorption of polycations and polyanions.1 Operationally, this method occurs through simple, consecutive exposures of a substrate to polycation and polyanion solutions, with rinsing to remove unadsorbed polymer after each deposition step. Typical polyanions employed for deposition of these films include ionized forms of poly(acrylic acid) (PAA), poly(styrene sulfonic acid) (PSS), and poly(vinyl sulfonic acid), whereas most polycations contain quaternary ammonium functionalities or protonated amines.2 In addition, early studies showed that LbL methods can also use a much broader range of multiply charged species, including proteins, viruses, nanoparticles, and exfoliated inorganic materials.2 In some cases, LbL methods also employ other interactions such as hydrogen bonding or covalent linkages.3
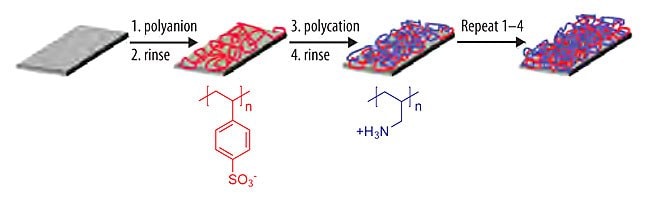
Figure 1.Schematic diagram of layer-by-layer adsorption of polyelectrolyte multilayers. The figure also shows the structures of two common polyelectrolytes, poly(styrene sulfonate) and protonated poly(allylamine).
The LbL strategy has a number of advantages over most thin film forming methods. First, this technique offers control over film thickness at the nanometer scale, because a single adsorption step can deposit as little as a few angstroms (Å) of polymer.3 Second, conformal adsorption occurs on substrates with a wide range of geometries, allowing coating of 3-dimensional objects such as nanoparticles and porous membranes (see below).4,5 Finally, the broad range of materials suitable for LbL adsorption and the ability to deposit species in a defined order can afford a wide variety of functional films. (We should note, however, that polyelectrolytes are frequently intertwined over several layers.1) Postdeposition reactions such as cross-linking and reduction of metal ions to form nanoparticles provide further ways to modify film properties. Prior studies of post-deposition reactions yielded functional films for catalysis,6 corrosion prevention, anti-reflective coatings, optical shutters, and superhydrophobic coatings.
Film Formation
The key feature in the deposition of polyelectrolyte multilayers (PEMs) is charge overcompensation. The initial layer adsorbs onto the substrate by electrostatic or hydrophobic interactions and either creates a charged surface or reverses the substrate surface charge. Adsorption of subsequent layers again overcompensates the charge on the surface to reverse the substrate′s charge and allow adsorption of the next layer.7 In many cases, the thickness of multilayer polyelectrolyte films increases linearly with the number of adsorbed layers. This suggests that the extent of charge overcompensation does not vary greatly with the number of adsorbed layers, so the amount of polyelectrolyte deposited in each step is approximately constant. However, for some polyelectrolyte systems, film thickness increases exponentially with the number of layers. Schaaf et al. suggest that the exponential growth occurs when one of the polyelectrolytes diffuses “into” the entire film during deposition.8 Upon addition of the oppositely charged polyelectrolyte, the previously adsorbed polyelectrolyte diffuses “out” of the whole film to form a very thick polyanion-polycation complex at the surface. Because one of the polyelectrolytes, usually one with low charge density and high swelling in water, diffuses into the entire film, the thickness of each adsorbed layer increases with the number of layers.9
In addition to the polyelectrolyte selected for deposition, a number of adsorption parameters such as supporting electrolyte concentration and composition, pH of the polyelectrolyte solutions, adsorption time, and temperature also influence the amount of polyelectrolyte deposited in LbL methods. Many studies show a dramatic effect of supporting electrolyte concentration on the thickness of PEMs. In the absence of added salt, polyelectrolytes are highly extended to maximize the distance between charged repeat units of the polymer. Under these conditions, adsorbed layers are thin and overcompensate the surface charge only slightly (Figure 2). For example, the thickness of a 10-bilayer PSS/poly(diallyl dimethyl ammonium chloride) film prepared without addition of salt is about 60 Å.10 Thus, the average thickness per layer is only 3 Å. However, when deposited from solutions containing 2 M salt, the thickness of the corresponding 10-bilayer film is more than 3,000 Å.10 Of course the film structure and composition also vary greatly with deposition conditions.
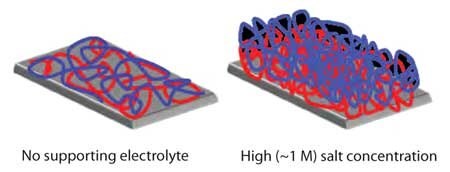
Figure 2.Schematic drawing of polyanion/polycation bilayers prepared without (left) and with (right) supporting salt.
Film Permeability
Studies of film swelling and transport through polyelectrolyte multilayers demonstrate the wide range of structural variation available with these coatings. Remarkably, chitosan/hyaluronic acid films swell by 400% in water. Accordingly, these films are permeable to molecules as large as myoglobin (17 kDa).11 In contrast, PSS/protonated polyallylamine (PAH) coatings swell by <100%, and these films can prevent transport of molecules as small as glucose. In general, coatings prepared from polyelectrolytes with a high charge density have a high degree of ionic cross-linking that leads to decreased permeability of small molecules along with relatively high transport selectivities between different molecules.12
Functionalized Membranes
PEMs are also attractive for coating surfaces with functional particles such as enzymes and catalysts. We are particularly interested in creating functionalized membranes (Figure 3) because they provide a relatively high surface area, and convective flow rapidly brings reactants to catalytic sites.13 The micron-sized pores lead to minimal diffusion limitations, and variation of flow rates can limit the residence time in the membrane to control the extent of reaction.
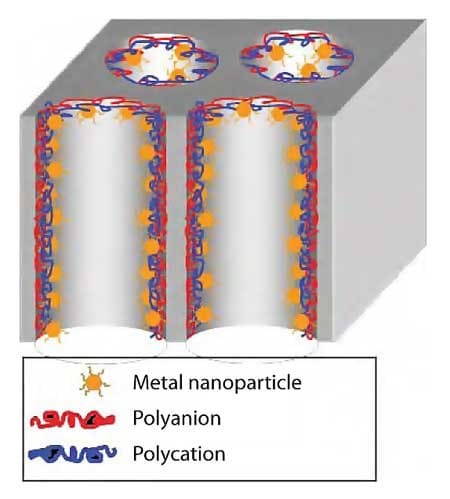
Figure 3.Schematic drawing of catalytic metal nanoparticles immobilized in a porous membrane using LbL deposition. Used by permission of the American Chemical Society.
LbL adsorption in membrane pores occurs by simply flowing polyanion, polycation, and rinsing solutions through the membrane. When citratecoated metal nanoparticles serve as the polyanion, this technique yields a high density of well-separated nanoparticles within membrane pores, as Figure 4 shows.14 This adsorption can occur in inorganic and polymeric flat sheet membranes as well as in polymeric hollow fiber membranes.14-16 Avoiding particle aggregation is important to maintain both a high catalytic surface area and the unique electronic properties of nanoparticles.
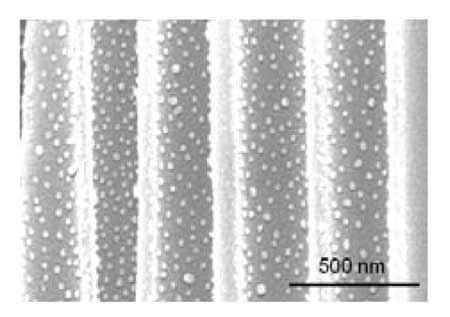
Figure 4.Cross-sectional SEM images of a porous alumina membrane coated with a PAA/PAH/Au nanoparticle film. The gold nanoparticles were stabilized by citrate. Used by permission of the American Chemical Society.
Remarkably, the catalytic activity of the nanoparticles in LbL membrane coatings is essentially the same as that of nanoparticles in solution. Moreover, control over the flow rate through the membrane can alter product distribution. For example, reduction of nitrobenzene by NaBH4 (Scheme 1) gives 24% nitrosobenzene and 73% aniline when the flux through the membrane is 0.015 mL/(cm2s), but 47% nitrosobenzene and 49% aniline result from a ten-fold higher flux.14 During the reduction step, less time in the membrane leads to higher amounts of the more valuable nitrosobenzene. Additionally, varying the flow rate reveals that nitrosobenzene is an intermediate in the reduction of nitrobenzene to aniline. Higher flow rates may yield even more nitrosobenzene, but a limit will eventually be reached and much of the starting material will remain unreacted.
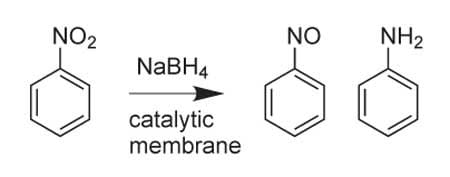
Scheme 1.Reduction of nitrobenzene to nitrosobenzene and aniline.
LbL methods can also be used to immobilize enzymes in membranes.17,18 In some cases, electrostatic interactions with the polyelectrolytes help stabilize the enzymes to maintain their activity. A few studies focused on LbL immobilization of trypsin in microfluidic chips to create systems that digest proteins prior to analysis by mass spectrometry.19 However, in these chips, diffusion distances can reach up to 100 μm, which may limit digestion rates. In membranes, the diffusion distances are often <1 μm, so more complete, rapid digestion should be possible. We employed adsorption of only 1 layer of PSS and trypsin in nylon membranes (nominal 0.45 μm pore size) to construct membrane reactors that rapidly digest proteins.18 This LbL process leads to deposition of ~11 mg of trypsin per cm3 of membrane pores, which is about 450 times greater than the typical trypsin concentration in solution-based protein digestion. In-solution digestion employs low concentrations of trypsin to avoid self-digestion of the enzyme, whereas in the membrane immobilization limits self-digestion.
The high concentration of trypsin in membranes modified using LbL deposition allows rapid, efficient protein digestion for mass spectrometry (MS). After digestion of α-casein in membranes, gel electrophoresis shows no residual protein even with residence times in the membrane as low as 0.8 seconds. Moreover, in matrix-assisted laser desorption/ ionization-MS (MALDI-MS), membrane digestion leads to signals for 52 proteolytic peptides, whereas solution digestion reveals only 37 peptides. The larger number of peptides that result from membrane digestion leads to a high amino acid sequence coverage (84%), which facilitates identification of protein modifications. Very short residence times in membranes, on the order of microseconds, can lead to much larger peptides, which may prove useful in protein identification or structural studies.
Future Opportunities
In the laboratory, LbL deposition is extremely convenient and versatile. However, for films containing many layers, the technique becomes time consuming and cumbersome, especially from a manufacturing standpoint. Rinsing steps also produce waste streams that must be treated or recycled. Thus, although LbL deposition of polycations and polyanions began in earnest 20 years ago, practical applications of these films have not rapidly followed. Efforts to prepare coatings by spraying may simplify the LbL process,20 but these methods may also sacrifice some control over resulting film structures. Future LbL applications may continue to expand by coupling nanotechnology to the process and lead to new innovations in sensors, where the film provides a unique function. A wide array of unique small-scale, functional and multifunctional coatings are possible.
Acknowledgment
We are grateful to the Department of Energy Office of Basic Energy Sciences and the National Institutes of Health for support of this work.
For a complete list of available polymers, visit our website.
Materials
References
To continue reading please sign in or create an account.
Don't Have An Account?