Polymersomes for Drug Delivery
Joe Collins, Ayana Bhaskaran, Luke Andrew Connal
Department of Chemical Engineering,Melbourne School of Engineering The University of Melbourne, Parkville 3010 Victoria, Australia
Material Matters, 2017, 12.2
Introduction
The development of drugs that target specific locations within the human body remains one of the greatest challenges in biomedicine today. The majority of currently administered drugs have no means of targeting specific tissues or cells. Non-specific drug activity on healthy cells can lead to serious side-effects, drastically reducing a patient’s quality of life. For example, chemotherapy typically leads to severe side effects such as hair loss, loss of the lining of the gut, ulcer formation, nausea, and more. Specific drug targeting has the potential to reduce or eliminate side effects and allow for a reduction in the dosage required, thus decreasing cost while increasing therapeutic efficiency and improving the quality of life. Although modern methods for drug delivery have improved selectivity, significant problems such as drug degradation, low bioavailability, and limited circulation times remain. An ideal drug delivery system should offer stability, targeting to a specific site within the body, and controlled release upon delivery to the target site.
Polymersomes, colloidal-sized hollow spheres comprised of an aqueous core surrounded by a polymeric bilayer membrane, are promising candidates for next generation drug delivery systems (Figure 1). Inspired by nature, polymersomes are synthetic analogs to liposomes found in all living cells. Formed by the self-assembly of amphiphilic molecules, polymersomes are capable of transporting hydrophilic molecules (loaded within the aqueous core of the polymersomes), hydrophobic molecules (loaded within the membrane bilayer), or a combination of both—allowing a greater therapeutic action than a single drug alone (Figure 1).1 Polymersomes feature enhanced toughness, reduced membrane permeability,2 and possess little to no immunogenicity (if designed correctly).3 These block copolymer vesicles can achieve targeted drug delivery through surface functionalization with ligands for specific cell receptors (e.g., proteins, carbohydrates, or small molecules). The controlled drug release from within polymersome capsules can be achieved through the incorporation of stimuli-responsive chemistry. Due to these benefits, polymersomes have been extensively explored in various biomedical applications such as drug delivery,3,4 gene and protein delivery,5 imaging,6 and diagnostics.7 This article will focus specifically on polymersomes for drug delivery.
Polymersome synthesis will be discussed followed by a review of surface functionalization to achieve polymersome targeting and the incorporation of dynamic or stimuli-responsive chemistry for controlled drug release.
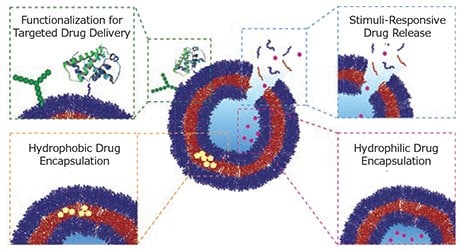
Figure 1. Schematic highlighting the advantages of polymersomes for drug delivery. Surface functionalization of polymersomes with carbohydrates, proteins, or small molecules allows for polymersome targeting to specific locations within the body (green box). Stimuliresponsive drug release allows for controlled drug delivery (blue box) of hydrophilic drug molecules, loaded within the polymersomes core (pink box) and hydrophobic drug molecules, loaded within the polymersome membrane bilayer (orange box).
Fabrication Process: Design, Synthesis and Drug Encapsulation
The development of controlled radical polymerization (CRP) techniques, such as nitroxide-mediated polymerization (NMP), reversible addition-fragmentation chain transfer polymerisation (RAFT), and atom-transfer radical polymerisation (ATRP), has allowed for the synthesis of well-defined, amphiphilic polymers (for example, PS-b-PAA, Figure 2A). Through CRP, polymers with precise molecular weights, low dispersity (Đ), and specific architectures can be synthesized. Examples include block copolymers (BCPs), triblock copolymers, and graft polymers. A wide range of amphiphilic polymers have been successfully synthesized through CRP and shown to self-assemble into interesting structures such as spheres/micelles, cylinders, and polymersomes.8
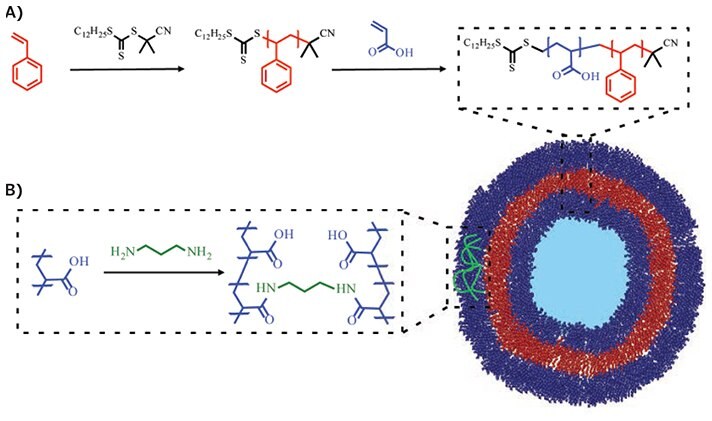
Figure 2. Representative synthesis of BCP via RAFT polymerization and BCP self-assembly into polymersomes, as well as polymersome stabilization through crosslinking. A) A hydrophobic block of polystyrene (PS) is produced by RAFT polymerization. From this is grown a hydrophilic polymer block of poly(acrylic acid) (PAA). Self-assembly of the PS-b-PAA BCP yields polymersome capsules. B) Crosslinking can be utilized to increase the stability of polymersomes represented here by amide formation between poly(acrylic acid) chains.
The self-assembly of BCPs in solution is governed by the packing parameter (p). The packing parameter can be used to indicate what structure will be formed upon self-assembly by taking into account the volume of the hydrophobic chain (v), the interfacial area per molecule (a), and the length of the hydrophobic chain (l) (Eq. 1). Different structures fall between different p values; if p<1/3 spherical structures will form, between 1/3<p<1/2 cylinders will form, and 1/2<p<1 polymersomes will form.

Equation 1. Packing parameter of amphiphilic BCPs.
The synthesis of polymersomes usually involves the slow addition of a select solvent to a dissolved solution containing the amphiphile. As the solvent properties change, amphiphiles selfassemble to minimize unwanted solvent-polymer interactions. A number of techniques have been developed for the preparation and characterization of polymersomes.8
Since only non-covalent forces govern polymersome assembly and stability, these supramolecular structures are inherently unstable, which is amplified upon introduction to areas of massive dilution, such as the blood stream. The stability of polymersomes can be increased by covalently crosslinking the hydrophobic core, hydrophilic shell, or core-shell interface. Crosslinking has been reported using diverse chemistries including amide, disulfide, UV-irradiation, and carbodiimide coupling. These and other means of polymersome crosslinking have been reviewed previously.3,4
Polymersomes have attracted great interest for drug delivery applications due to high drug loading capability and the ability to carry both hydrophilic and hydrophobic molecules. Hydrophobic molecules integrate into the non-aqueous membrane bilayer and hydrophilic molecules are captured inside the aqueous core. A wide range of hydrophobic and hydrophilic molecules have been successfully loaded into polymersomes.3,4 Specific examples include membrane proteins, hydrophobic drugs (e.g., Paclitaxel), hydrophobic molecules (e.g., camptothecin, Product No. C9911), and hydrophilic drugs (e.g., Doxorubicin hydrochloride [DOX HCl], Product No. D1515). The ability to load both hydrophobic and hydrophilic molecules simultaneously into polymersomes often results in far greater therapeutic effect than a single drug alone. A report by Thambi et al. describes the synthesis of a redox-responsive polymersome composed of the triblock copolymer PEG-b-PLys-SS-PCL which was successfully loaded with DOX HCl (in the aqueous core) and camptothecin (in the membrane bilayer). Higher cytotoxicity was reported when the dual-drug loaded polymersome was utilized in comparison to a single administration of either drug alone.1
In general, drug release from polymersomes occurs through passive diffusion, driven by concentration gradients. The rate of diffusion can be tuned through modification of the bilayer membrane thickness, covalent crosslinking, and control over amphiphile composition. To overcome problems associated with non-specific drug release, many stimuli-responsive polymersomes have been developed to achieve controlled drug delivery at target locations.
Functionalization for Targeted Drug Delivery
Polymersomes for targeted drug delivery feature surfaces functionalized with specific targeting groups and/or ligands. A range of molecules have been explored as targeting ligands (Table 1).
The conjugation of targeting molecules onto the surface of polymersomes can be achieved in two ways. In the first method, the targeting ligand is conjugated to active groups presented on the polymersome surface after polymersome formation (Figure 3A). Alternatively, the targeting ligand is conjugated onto the amphiphilic polymer prior to polymersome formation. The functionalized polymer is then allowed to assemble into the final polymersome structure with the targeting ligand on its surface (Figure 3B). Both approaches are feasible for small molecules and peptides; however, larger molecules such as polysaccharides and proteins, must be incorporated postpolymersome formation, as they can act to disrupt the selfassembly of copolymer molecules into polymersomes.
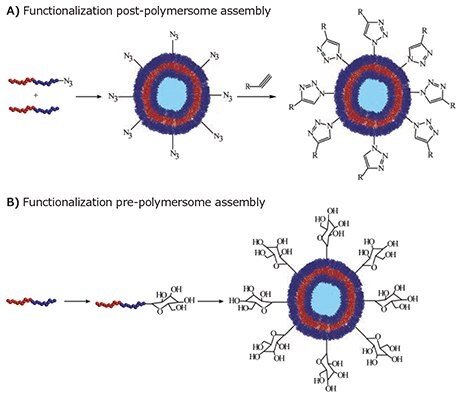
Figure 3. Strategies for the incorporation of targeting groups onto a polymersome surface. A) Targeting groups can be added to reactive groups presented on the polymersome surface post-polymersome formation. This process is exemplified here through the conjugation of an alkynefunctionalized targeting group onto azide groups presented on a polymersome surface. B) Alternatively, the targeting group can be conjugated onto the amphiphilic polymer pre-polymersome formation. The functional polymer is then self-assembled into the final polymersome structure which displays the targeting groups on its surface. This process is exemplified here through the conjugation of a sugar molecule onto a block copolymer, which is then selfassembled into a polymersome displaying the sugar groups on its surface.
Several attempts have been made to conjugate ligands on preformed polymersomes using click chemistry.20,21 Due to their high efficiency and orthogonality, click reactions are an effective method to achieve a high degree of surface functionalization. For example, van Hest and coworkers synthesized polymersomes composed of an azide-functionalized amphiphilic polystyreneblock- poly(acrylic acid) (PS-b-PAA-N3) copolymer.20 The coppercatalyzed azide-alkyne (CuAAC) reaction was then used to bind an alkyl-bearing fluorescent dansyl probe and/or biotin onto the polymersome surface, allowing for effective surface functionalization.20 Martin et al. also used the CuAAC reaction to conjugate alkyne-functional dendritic and non-dendritic mannose derivatives onto polymersomes comprised of azide-functional poly(butadiene-block-ethylene oxide-N3) BCP.21 The dendritic derivatives displayed a two-fold higher binding affinity towards concanavalin A than the non-dendritic derivatives.21
As mentioned previously, targeting ligands can be grafted onto the end of the amphiphilic BCP prior to polymersome formation. As the ratio of functional to non-functional BCP can be controlled, the surface density of the targeting ligand in the final polymersome assembly can also be easily tuned. This is an advantage over functionalization post-polymersome formation, in which control over the degree of surface functionalization is much more limited. Utilizing the prepolymersome functionalization strategy, Kim et al. successfully managed to target E. coli bacteria with a mannose-functionalized tetra(p-phenylene)-block-PEG copolymer. The self-assembled polymersomes were reported to display an 800-fold increase in the binding affinity towards E. coli pili compared to nonfunctionalized polymersomes.22
Significant efforts have been made to improve the potency of chemotherapy by targeting the active drug to the tumor site. Polymersomes have been successfully used to target different sites within the body, such as the central nervous system (CNS), brain, cochlea, and macrophages (Figure 4).8 Certain cell surface receptors are over expressed in human cancer cell lines including breast, prostate, colorectal, and lung cancer cells.3,8 This can be exploited for polymersome targeting though the conjugation of specific binding ligands onto the polymersome surface. Alternatively, many cancers overexpress certain enzymes. Careful design of block copolymers, in which the polymer blocks are linked via enzyme cleavable groups, enables specific drug release once the polymersome has entered the target cell. Jung et al. developed biodegradable polymersomes from methoxy poly(ethylene glycol) and poly(d,l-Lactide) diblock copolymers, with a connecting peptide sequence Gly-Phe-Leu- Gly-Phe (GFLGF) that is cleavable by lysosomal enzymes present in tumor cells.14 Antibody-mediated endocytosis followed by the cleavage of the peptide sequence in the resulting polymer (mPEG-GFLGF-PDLLA), leads to dissolution of the polymersome and concurrent drug release.
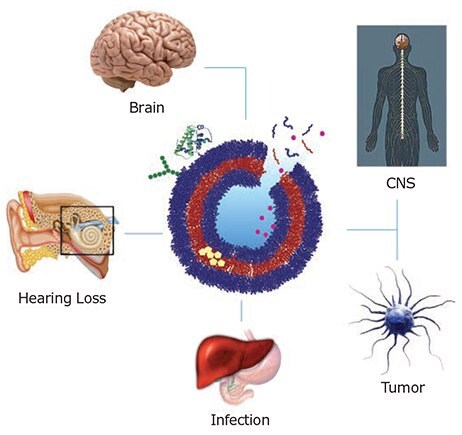
Figure 4. Functionalized polymersomes are used for diagnostic and therapeutic applications. Targeted drug delivery to various organs have major research focus on neurodegenerative diseases, cancer treatment, sensorineural hearing loss, infection and inflammation detected in the CNS, brain, tumor cells, cochlea, and macrophages, respectively.
Once polymersomes are targeted to specific locations, the encapsulated drug must be released to attain a therapeutic effect. Controlled drug release can be achieved through the incorporation of stimuli-responsive chemistry into the polymersome structure.
Responsive Drug Release
By incorporating a variety of dynamic or responsive chemistries into the polymer building blocks, polymersomes can be designed to release their payload upon the application of a diverse range of stimuli. The ability to trigger drug release at target locations allows for superior drug activity and the minimization of unwanted side effects. In general, stimuliresponsive drug release from polymersomes is achieved through three mechanisms: cleavage of a stimuli-responsive group linking the polymer blocks (Figure 5, red), degradation of the intermolecular bonds between monomer units in a polymer block (Figure 5, blue), or through an electrostatic change in one of the polymer blocks resulting in a shift from a hydrophobic to hydrophilic state (Figure 5, green). All three mechanisms result in the dissolution or degradation of the polymersome and release of the encapsulated cargo.
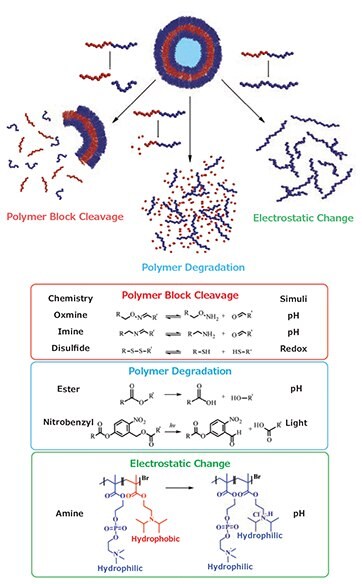
Figure 5. Degradation/dissolution of polymersomes by polymer block cleavage (red), polymer degradation (blue), or through an electrostatic change (green).
Many examples of stimuli have been reported to achieve drug release from polymersomes such as pH, redox, reaction with reactive-oxygen species or glucose, or through the application of external stimuli such as a magnetic field, ultrasound, or light. Several recent comprehensive reviews have described the synthesis and applications of stimuli-responsive polymersomes.3,4 The three most researched stimuli are pH, redox potential, and light. Two rely on naturally occurring physiological gradients (pH and redox potential), while the other relies on the application of an external stimulus (light).
The development of pH-responsive polymersomes has received significant attention due to naturally occurring pH gradients in the body. Physiological pH is balanced around pH 7.4, with lower pH environments occurring in inflammatory and tumor tissue (pH 6.5–7.2) and inside lysosomes and endosomes (pH 4.5– 5.5). This provides a facile means to achieve polymersome degradation and drug release through the incorporation of pH-sensitive or ionizable bonds in the amphiphilic polymer.
Block copolymers with a hydrophobic block comprised of a hydrolytically degradable polyester, such as poly(lactic acid) (PLA) or poly(ε-caprolactone) (PCL), were some of the earliest pH-sensitive polymersomes developed.23 Polymersome dissolution (and drug release) is achieved through hydrolysis of the ester bonds linking the polymer blocks. PEG-b-PCL and PEGb- PLA polymersomes, loaded with DOX or Paclitaxel, were shown to localize in tumor tissue in mice and release the encapsulated payload upon entering the acidic tumor environment, arresting tumor growth and yielding tumor shrinkage.24
An alternate method of pH-stimulated drug delivery from polymersome vesicles, involves linking the polymer blocks through a pH-sensitive bond, such as an imine, hydrazone, or acetal.4 Upon exposure to acidic conditions, the link between the hydrophilic and hydrophobic block degrades, leading to the breakdown of the polymersome and release of the encapsulated cargo.
pH-triggered polymersome degradation can also be obtained through the incorporation of an ionizable group into one of the polymer blocks. Examples include the synthesis of a zwitterionic poly(2-(methacryloyloxy)ethylphosphorylcholine)-b-poly(2- (diisopropylamino)ethyl methacrylate) (PMPC-b-PDPA) block copolymer prepared by Armes and co-workers.25 The PMPC-b- PDPA polymersome was designed to be stable at physiological pH, but degradable under acidic conditions. Degradation was achieved through the protonation of the tertiary amine groups of PDPA, which prior to protonation is hydrophobic but becomes hydrophilic upon protonation, resulting in polymersome dissolution. Due to the acidic pKa of the amine (6.4) in PDPA, the polymersomes are stable in the bloodstream at physiological pH (7.4) but degradable under the acidic conditions found in lysosomes and endosomes.
Redox active polymers are a versatile platform for targeted drug delivery, as redox potentials vary greatly between normal and tumor tissue, the intracellular and extracellular environment, and even between different cell organelles. Glutathione (GSH), an important cellular reducing agent, is often used to trigger the reduction of sensitive linkages for polymersome disassembly. GSH concentrations are typically very low in plasma and normal tissue (2–20 μM) but significantly higher in the cytosol, nuclei, and tumor tissue (2–20 mM), producing a high intracellular redox gradient. Disulfide (-S-S-) bonds are susceptible to reduction by GSH, to produce two thiol (-SH) groups. This has been exploited for target drug delivery by incorporating disulfide bonds into the polymer backbone of polymersomes, such as in the synthesis of the triblock copolymer PEG-MA-b-PCL-S-S-PCL-b-PEG-MA26 or as a chemical crosslinker as in the case of a PEG-b-PLys-b- PCL polymersome.27 GSH reduction and subsequent disulfide cleavage leads to polymersome disassembly and the release of the encapsulated payload.
Light is an attractive means of triggering drug release, as it allows for a high degree of temporal and spatial control, and uses a non-invasive method. Using light to trigger drug release from polymersomes is usually achieved either through a structural change in the polymersome or through polymer degradation through light irradiation. A variety of light-sensitive groups have been introduced into polymersomes such as spiropyran, 2-nitrophenylalanine, and o-nitrobenzyl; these have been reviewed previously by Hu et al4
Conclusion
The unique charateristics of polymersomes make them highly promising materials for drug delivery applications. We have summarized the design and fabrication considerations for polymersomes, highlighting drug encapsulation procedures. The ability to functionalize the polymersome surface for active targeting and for controlled drug release demonstrates the power of these versatile nanomaterials.
Related Products
References
To continue reading please sign in or create an account.
Don't Have An Account?