Organic Bioelectronics
Bryan D. Paulsen1, 2, Alexander Giovannitti3, 4, Vishak Venkatraman1, 2, Jonathan Rivnay1, 2*
1Department of Biomedical Engineering, Northwestern University, Evanston, IL 60208, US, 2Simpson Querrey Institute for BioNanotechnology, Northwestern University, Chicago, IL 60611, USA, 3Department of Chemistry, Imperial College London, London SW7 2AZ, UK, 4epartment of Physics and Center for Plastic Electronics, Imperial College London, London SW7 2AZ, UK
Material Matters, 2019, 14.1
Article Sections
- Introduction
- Conjugated Polymeric Mixed Conductors
- PEDOT:PSS Based Bioelectronics
- Glycolated Conjugated Polymers: Beyond PEDOT:PSS
- Conclusion
Introduction
Bioelectronics, the convergence of biology and electronics, seeks to leverage microelectronics to sense, stimulate, and control biological systems. Biological systems employ complex molecular and cellular mechanisms to propagate signals through the motion and accumulation of ionic charges (anions and cations). Traditional microelectronics rely on metals, inorganic semiconductors, and oxide dielectrics to propagate signals through transport and accumulation of electronic charges (electrons and holes), leading to a fundamental mismatch in signal transmission. Organic bioelectronic materials have significant advantages over traditional semiconductors and metals, making them superior candidates for next generation interfaces with biological systems.1,2 Organic materials have proven especially effective at interconverting ionic and electronic signals, thus efficiently bridging the ionic world of biology with contemporary microelectronics. Further, organic materials can be solution processible, can form soft, flexible devices that conform to the complex curvilinear surfaces of living organisms, and can decrease the mechanical mismatch between electronic devices and the surrounding biological material. Herein we review the recent advances in organic bioelectronics, highlighting new materials and applications.
Conjugated Polymeric Mixed Conductors
Within the larger field of organic electronic materials, there exists a subset of mostly polymeric materials that are mixed conductors (both ionic and electronic), making them especially powerful for bioelectronic applications. These conjugated polymeric mixed conductors can efficiently transport charge through both the transport of holes and/or electrons (electronic conduction) and via mass transport of ionic species (ionic conduction). The transport of electronic charge in these materials occurs via hopping along and between the π-conjugated backbones of the polymer chains. Ionic transport occurs via the intercalation and drift or diffusion of ions in the void spaces between the polymer chains, with solvent swelling of polymers often increasing the ease of ion motion. However, simultaneous ionic and electronic transport is not sufficient for bioelectronic applications. Crucially, there must also exist strong ionic-electronic coupling such that ionic currents can induce electronic currents and vice versa. In conjugated polymeric mixed conductors, this occurs via the redox process of electrochemical doping, where ionic species stabilize mobile electronic charges along the polymer backbone. In bioelectronic devices, this ionic-electronic coupling manifests itself as conversion, or transduction, from biological ionic signals to electronic signals.
Organic mixed conductors are especially efficient ionic-electronic transducers and interact with biological systems in a threedimensional manner, an improvement over traditional metal or semiconductor materials that only interact with a biological system across a two-dimensional interface (Figure 1A). Polymeric mixed conductors can swell with and access ions and water molecules from the biological medium throughout the entire volume of the polymer, thus enabling a 3-dimensional interfacing surface area (Figure 1B). The permeable polymer presents no ionic barrier at the material-electrolyte interface, massively increasing the effective interfacial area while avoiding the many complications that occur at discrete solid-electrolyte interfaces such as native oxides, dangling bonds, corrosion, and surface reorganization. Given this, an important figure of merit for mixed conducting polymers is the volumetric capacitance, C*, which is a measure of the magnitude of ionic-electronic coupling per unit volume. This capacitance is critical because the magnitude of the ion-electronic coupling directly determines the strength of ionic to electronic signal transduction.3 Given that mixed conducting polymers have high accessible surface areas and large volumetric capacitances (in contrast to traditional microelectronic materials’ limited surface areas and low volumetric capacitances), biological probes fabricated from polymers can have orders of magnitude higher capacitance, lower interfacial impedance, and thus vastly improved signal transduction compared to identical volume metal or semiconductor probes.
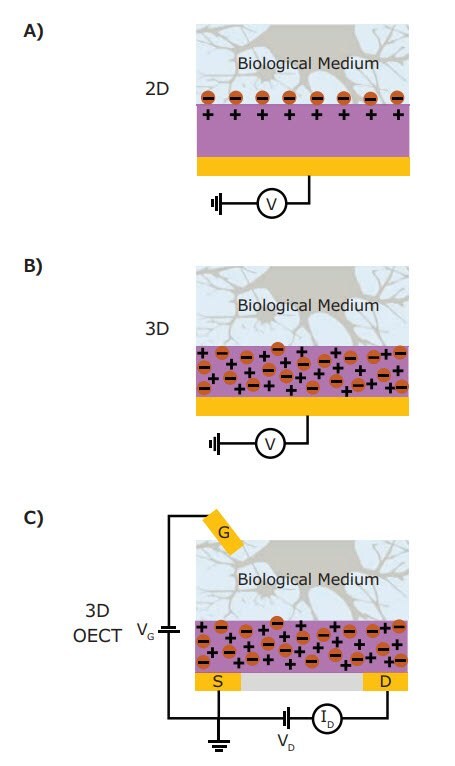
Figure 1. Schematic diagram of the various bioelectronic interfaces. A) A two-dimensional high impedance interface at an ion-impermeable electrode. B) A low impedance three-dimensional interface in a mixed conductor electrode. C) The same mixed conductor incorporated into organic electrochemical transistors (OECT).
Beyond the volumetric effect, conjugated polymer mixed conductors can be employed as the channel material in devices for biological recordings, such as organic electrochemical transistors (OECT). Transistors are electrical switches where a semiconductor is modulated between a conductive (on) state and a resistive (off) state. In OECTs, the electrochemical doping mechanism that allows for efficient ionic to electronic current transduction also modulates the electrical conductivity of the conjugated polymer. The strength of this effect, the change in conductivity with change in volumetric charge density, is represented as a transconductance (gm). In an OECT architecture interfaced with a biological environment, the electrical conductivity of the mixed conductor film can be monitored rather than the potential by harnessing the transconductance. By appropriately biasing the OECT, the change in conductivity induced by the biological signals can be large as determined by the device transconductance, effectively amplifying the biological signals (Figure 1C). This allows a single mixed conducting material to simultaneously perform efficient transduction and amplification of biological signals directly at the biotic-abiotic interface.
PEDOT:PSS Based Bioelectronics
By far the most widely employed polymeric mixed conductor in organic bioelectronics research has been PEDOT:PSS. Extensively employed in antistatic coatings and organic photovoltaic (OPV) research for over two decades, PEDOT:PSS (Cat. No. 900181, 655201, 739324) is a blend of the conjugated polymer poly(3,4- ethylenedioxythiophene) (PEDOT) and the polyelectrolyte poly(styrenesulfonate) (PSS). A fraction of the PSS repeat units are deprotonated, and the resulting negatively charged sulfonate units stabilize holes on the PEDOT, effectively doping it to produce an electrically conductive composite. PEDOT:PSS and other PEDOT-based inks (Cat. No. 900442, 901047, 675288) are typically formulated as dispersions; when cast in a film, the materials exhibit conductivities in the range of 10–1,000 S cm-1 depending on formulation and processing additives. In the thin film state, PEDOT:PSS forms a microscale morphology of intermixed PEDOT-rich and PSS-rich phases. PSS readily swells upon exposure to water, thus providing efficient pathways for ion transport within PEDOT:PSS films. Due to the high miscibility of PSS with water, crosslinking agents are often necessary to prevent redispersion and maintain the film stability. While the extensive use of PEDOT:PSS is in part due to its commercial availability, PEDOT-based materials synthesized via electropolymerization and vapor phase polymerization have also been effectively employed in bioelectronic applications.
PEDOT-based materials have been incorporated into a wide variety of devices and applications, of which only a brief selection can be covered here (Figure 2). For measurement and recording of biological signals, PEDOT:PSS incorporated in OECT structures has been employed in both high quality electroencephalography (EEG)4 and in conformal arrays in subcranial electrocorticography (EGoG).5 Biological growth has been measured by employing PEDOT:PSS cell scaffolds to measure cell growth, motility, or coverage via impedance changes.6 In addition to measuring biological activity, PEDOTbased materials can also control and stimulate biological activity. When used as a cellular substrate, the redox state of PEDOT:Tosylate can be tuned to control adhesion and density of epithelial and neural stem cells.7,8 In an OECT configuration, PEDOT:PSS can depolarize local neuronal membranes generating action potentials. Additionally, these PEDOT:PSS OECTs can be fabricated on delaminating flexible parylene probes that can reside in vivo for a month without glial scarring, decreasing their stimulating electrode efficacy due to the decreased mechanical mismatch between the probe and surrounding brain tissue.9
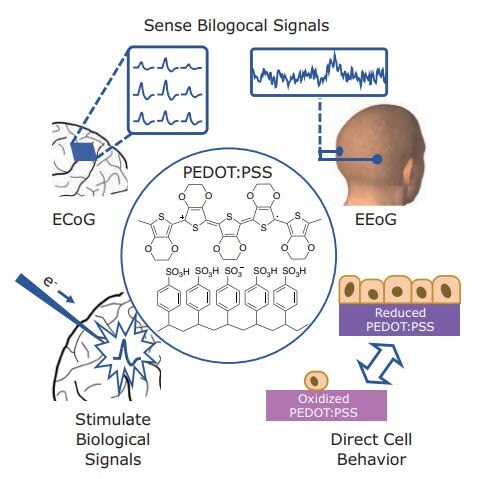
Figure 2.Structure of PEDOT:PSS and examples of applications in electrical recording and stimulation. Sensing biological signals via EGoG and EEG, stimulating local neuron action potentials by depolarization with electrical currents, and directing cell growth with redox switchable substrates.
When stimulating biological material, an electrical signal is transduced into an ionic signal, where the ionic currents are comprised of the ionic species present in the biological environment. In certain applications it is desirable to induce a current of a particular ionic species, especially in neurological applications where neuronal activity is gated by ion- or neurotransmitter-specific channels. With this application in mind, PEDOT:PSS has been used in organic electronic ion pumps.10 Ion pumps drive cations from a reservoir, through a cation exchange membrane (CEM) such as PSS (Cat. No. 243051, 434574) or poly(styrene sulfonate-co-maleic acid) (PSSA-co-MA), to a location of interest (Figure 3A). The geometry of the ion pump is determined by lithographic patterning, with the cation delivery location specifiable on the micron scale. In order to produce the driving potential, an electrode material is necessary to act as an oxidizable source within the cation reservoir and a reducible sink in the delivery environment, return reservoir, or waste.
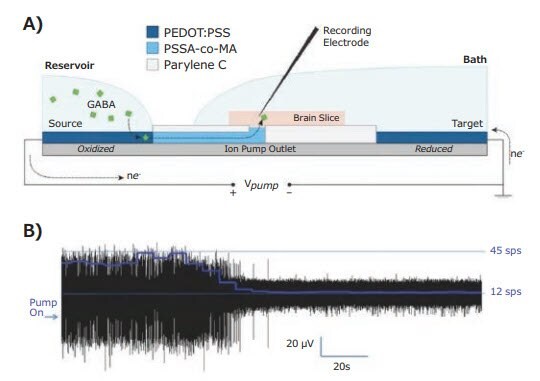
Figure 3. Chemical release with organic electronic ion pumps (OEIP). A) Schematic diagram of a PEDOT:PSS based ion pump performing local delivery of GABA inhibitory neurotransmitter and B) the typical response showing suppression of hyperexcited brain slice using OEIP GABA delivery.11
The volumetric capacity of PEDOT:PSS makes it an ideal redox electrode material for ion pumps, and has enabled the delivery of suitable ion concentrations to modulate neural activity.11 In particular, the suppression of epileptiform activity has been reported in hippocampal slices in vitro.11 PEDOT:PSS was able to deliver sufficient amounts of the inhibitor neurotransmitter λ-aminobutanoic acid (Cat. No. A2129), commonly known as GABA, to suppress epileptiform activity induced by a variety of techniques (Figure 3B). This showed direct ionic delivery of GABA as an apparent general method of suppressing epileptiform activity without the solvent or osmotic stressors associated with fluidic delivery. Further, ion pumpdriven GABA delivery was highly site specific, with no measured GABA inhibition in adjacent hippocampal areas. Ion pump delivery of GABA has been demonstrated in vivo, with ion pumps performing localized cortical and spinal cord delivery of GABA in living animal test subjects.12, 13 Along with these applications, PEDOT-based materials have contributed to advancements in artificial muscles (actuators),14 switchable surfaces,15 and biomimetic devices such as artificial synapses for neuromorphic computing devices.16
The combination of high electrical and ionic conductivity of PEDOT:PSS, along with its commercial availability, has led to wide application of PEDOT:PSS in the field of bioelectronics for organic materials. Despite its success, PEDOT:PSS has inherent properties that limit its application. First, PEDOT:PSS is exclusively a p-type material, meaning electronic charge transport occurs solely through hole conduction. In contrast, n-type materials are electron conductors, or ambipolar materials, that can support both hole and electron conductors. Further, PEDOT:PSS is naturally a depletion mode material, meaning it is in an electrically conductive state by default, and can be electrochemically switched into a non-conductive state. This is in contrast to accumulation or enhancement mode materials which are in a non-conductive state by default, but can be electrochemically switched into a conductive state. Thus, PEDOT:PSS is not a suitable material for the large number of applications that require n-type and/or accumulation mode materials.
Glycolated Conjugated Polymers: Beyond PEDOT:PSS
Due to the inherent limitations of PEDOT:PSS, sustained progress in the field of organic bioelectronics requires the development of new materials. By moving away from blends of separate ionic and electronic conducting polymers, recent successful approaches have resulted in single-component materials that efficiently perform both the electronic and ion transport functions. In electrically conducting polymers, backbone π-conjugation is required for efficient charge transport. Therefore, synthetic design routes to improve ion transport have recently turned to polymer side chain engineering. In the development of organic electronic materials, alkyl side chains were first added to conjugated polymers to increase their solubility in organic solvents and to improve processability. Eventually, side chain engineering became an effective route to direct polymer microstructure and morphology, thus controlling electronic properties. Due to the power of side chain engineering,17 work in this area has flourished.
To date, reported side chain engineering efforts to induce ionic conductivity have generally followed one of two routes. The first, more extensively studied route, employs side chains to tether ions to the polymer backbone, to create a conjugated polyelectrolyte.18,19 The second, more recently developed route, employs replacing the typically alkyl side chains of conjugated polymers with oligo-ethylene glycol side chains, consisting of repeating CH2-CH2-O structures (glycol side chains). These glycol side chains contain polar ether oxygens that readily coordinate to both ions and water, similar to the way solid polymer electrolyte polyethylene glycol behaves. Herein, we focus on the second route, which has produced most promising results of late.
Glycolated conjugated polymers for bioelectronics applications have been using the same synthetic tool box as cuttingedge polymers used in field effect transistor and photovoltaic applications. Monomers have been functionalized with polar glycol side chains prior to polymerization and polymerization was then carried out using C-C coupling reactions commonly used for conjugated polymers, such as Stille or Suzuki couplings. These methods have been used to produce a new family of glycolated conjugated polymers that are true single component mixed conductors, that are solution processible from common organic solvents, stable in aqueous conditions without crosslinking, operate as accumulation mode materials, and (depending on backbone chemistry) display either p- or n-type behavior.
While glycolated conjugated polymers for bioelectronics applications have only recently been reported, their advantageous properties have already enabled improvements in device performance and enabled new capabilities in bioelectronics. For example, two newly synthesized glycolated polythiophenes have reportedly achieved higher C* and transconductance gm than PEDOT:PSS in equivalent devices.3,20 In the case of poly(2-(3,3′-bis(2-(2-(2- methoxyethoxy)ethoxy) ethoxy)-[2,2’-bithiophen]-5-yl)thieno [3,2-b]thiophene), p(g2T-TT), the alkyl side chain equivalent polymers were synthesized and tested to show unequivocally the role the side chain plays in determining the advantageous ionic transport into the polymer film.21
As with PEDOT:PSS, the high performing p(g2T-TT) was found to be a p-type material, but in contrast to PEDOT:PSS, it operates as an accumulation mode material. This allowed for the first demonstration of an OECT biosensor operated in the subthreshold regime.22 In OECTs that function as true switches, the subthreshold regime is where the initial exponential increase in conductivity occurs when switching, via electrochemical potential, from the OFF (highly resistive) to the ON (highly conductive) state. By operating in this regime, sensing circuits incorporating p(g2T-TT)-based OECTs produced high voltage gain (biological signal transduction) with very low power consumption. This is important as low power consumption is required for implantable sensors and devices. It must be noted that the subthreshold regime is not accessible in PEDOT:PSS based devices, since the electrochemical potential required to switch off the conductivity of PEDOT:PSS exceeds the stability limits (electrochemical window) of water. The efficacy of the subthreshold regime for biological sensing was validated by measuring EEG signals. The OECT EEG sensor demonstrated two orders of magnitude improvement in signal strength as compared to commercial medical electrodes. Because p(g2T-TT) based OECTs operate in accumulation mode, they can truly turn off. Thus, they are suitable for use in multi sensor arrays, where individual sensing OECTs are turned on and “read” in sequence. Combined with their low power subthreshold operation, p(g2T-TT) OECTs present exciting prospects for next generation, low power conformable implantable biosensing arrays.
Using glycol side chains has led to the development of n-type conjugated polymer mixed conductors. In general, stable, efficient n-type electron transporting conjugated materials are less frequently reported than their p-type counterparts. In general, oxygen and moisture are incompatible with stable n-type behavior, thus complete fabrication and testing in inert glove box environments is often required. Because of this, applications requiring n-type materials have often relied on carbon nanotubes (Cat. No. 901046, 901056, 901082, 755125, 791490, 791504, 792462), metal oxides, or more exotic 2-D materials (Cat. No. 901940, 763705, 763713). So, it is very exciting that the design of n-type conjugated polymer mixed conductors produced materials that operate in aqueous electrolytes under ambient conditions with a high degree of stability. A series of n-type polymeric mixed conductors based on naphthalene diimide (NDI, 766038), bithiophene (T2, Cat. No. 241636, 577812, 733725, 515493), and alkoxybithiophene (gT2) repeating units has recently been reported.23,24 Interestingly, statistical copolymers containing 90% glycolated NDI units and 10% alkylated NDI units (Figure 4) have shown improved performance over all-gylcolated polymers. As with the p(g2T-TT) discussed above, these n-type polymers behave as accumulation mode materials as well.
To take advantage of n-type and accumulation mode behavior, the 90% glycolated copolymer (P-90) was applied as an electrochemical enzymatic sensor25 to detect charge transfer from an enzyme to a mixed conductor film as the target substrate was metabolized.
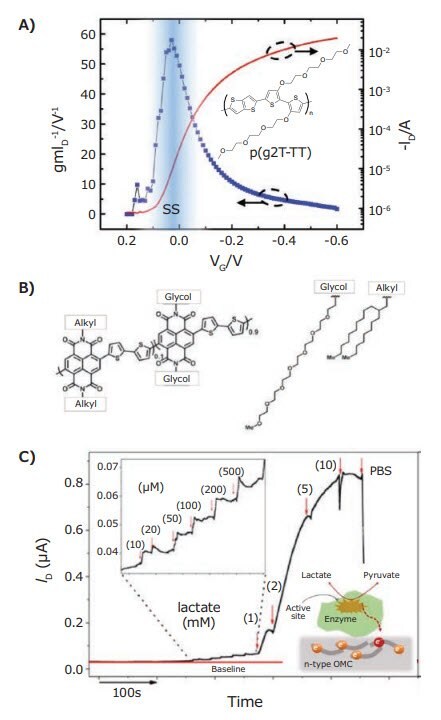
Figure 4. A) Transfer curve and normalized transconductance highlighting the subthreshold slope region of a p(g2T-TT) based p-type accumulation mode OECT,22 with inset p(g2T-TT) chemical structure. B) Chemical structure of n-type NDI 2T based statistical copolymer P-90. C) Lactate concentration dependent drain current (ID) of a P-90 based n-type accumulation mode transistor. Inset: Micro molar lactate sensitivity and a schematic diagram of electrochemical enzymatic mechanism of lactate concentration dependent drain current (conductivity) modulation.25
For such a charge transfer to occur, the mixed conductor must be redox active in the same range of electrochemical potentials as the enzyme. In the case of lactate oxidase, which undergoes a reduction at positive potentials, this requires a n-type polymeric mixed conductor. When converting lactate to pyruvate, the enzyme lactate oxidase (Cat. No. L9795) efficiently transferred charge to the P-90 copolymer. In turn, this charge transfer modulated the conductivity of P-90 in proportion to the concentration of lactate. By operating the sensor as an OECT, the local amplification of the enzyme charge transfer to P-90 allowed for micrometer scale sensors to sense lactate concentrations down to the micromolar level.
Conclusion
Organic materials are powerful tools in the arena of bioelectronics, due to their capability for mixed ionic and electronic conduction. Mixed conduction allows for vast improvements in both transduction and amplification of biological signals. Such advantages have been most widely exploited using PEDOT-based materials, especially the commercially available PEDOT:PSS. Recent developments of novel conjugated polymers with polar glycol side chains have produced significant performance gains over PEDOT:PSS, and have opened up new applications in electrochemical enzymatic sensing and low power electrophysiological measurements. Yet the field of conjugated polymer mixed conductors, especially those with glycolated side chains, remains relatively new, with plenty of novel materials and applications to be developed to raise the field of organic bioelectronics to the next level.
Materials
References
如要继续阅读,请登录或创建帐户。
暂无帐户?