Collagen in Gene Delivery Applications
Morgan A. Urello1, Kristi L. Kiick2, Millicent O. Sullivan1
1Dept. of Chemical and Biomolecular Engineering, University of Delaware, Newark DE 19716, 2Dept. of Material Science and Engineering, University of Delaware, Newark DE 19716
Introduction
Collagen-based delivery systems effectively deliver a range of viral and non-viral gene carriers by providing spatiotemporal control over delivery and reducing degradation rates and immune responses. The application of modified collagens has shown significant progress in enhancing bone and tissue repair, slowing tumor growth, and improving the overall efficacy and safety of gene delivery. Modified collagens have also facilitated controlled transgene expression in clinical trials.1 These advances are enabled by the biocompatibility and versatility of native collagen, which permits the incorporation of genes in collagen-based structures with a diverse range of properties. Furthermore, the development of modified collagens as well as synthetic collagen-mimetic peptides (CMPs) has provided additional control over gene delivery through alteration of vector/collagen affinity, modulation of cell/vector interactions, and increased vector stability.2,3 Overall, the unique structural properties and bioactivity of collagen and its derivatives coupled with the ease of tailoring its mechanical and degradative properties, and native cellular interactions, make collagen an enormously promising material for engineering high efficiency, tunable gene delivery systems.
Collagen as a Biomaterial
Collagen is the main structural protein in the extracellular matrix (ECM).1 It is located throughout skin and other connective tissues, where it provides the mechanical strength and structural integrity necessary for function. The unique shape and structural properties of collagen molecules are determined by their triple-helical α-domains. The triple helical domains in collagen consist of three polyproline chains composed of multiple triplets with the amino acid sequence Glycine-X-Y (GXY), in which X and Y are most commonly proline and hydroxyproline, respectively. Stabilized primarily by interchain hydrogen bonding the three polyproline chains associate together to produce a tightly packed triple helix.
Collagens play key roles in the cellular adhesion and signaling processes underlying development, tissue repair/regeneration, and cancer.1 As a biomaterial, collagen can similarly serve as both a bioactive, structural scaffold and a reservoir for retention and delivery of signaling molecules or genes. Collagen is biodegradable, non-immunogenic, and extremely versatile, and has been used to prepare a wide range of products in various forms. For instance, collagen has been used extensively as an injectable solution that spontaneously gels post-delivery to augment soft tissues, and as a porous, ECM-like sponge for the dressing of wounds.2
Modified Collagens and Collagen-Mimetic Peptides
Of the 28 types of collagen, type I collagen is the most common in nature and in biomaterial applications.1 Additionally, modified collagens have been developed to enhance collagen biomaterial properties and therapeutic gene delivery applications. Two of the most common collagen-derived biomaterials are atelocollagen and gelatin. Atelocollagen is obtained by pepsin treatment of collagen while gelatin is prepared by thermal denaturation of collagen following acid, alkali, or enzymatic pretreatment. Atelocollagen has similar structural properties to native collagen, yet with reduced immunogenicity due to the removal of the immunogenic telopeptides during enzymatic treatment. Gelatin similarly lacks significant antigenicity and can easily be crosslinked after chemical modification. Uniquely, the processing of gelatin can be used to vary its isoelectric point, which is valuable when forming polyelectrolyte complexes with other polymers or vectors. Additionally, like its collagen precursor, gelatin adheres extremely well to cells; however, intact versus denatured collagens present different integrin-binding sites, leading to significant differences in cell behavior. Alternatively, CMPs have been synthesized with a wide range of properties. These relatively short peptides consist of six to ten GXY triplets and have been engineered with various melting temperatures that can be altered by changing the length and/or composition of the amino acid sequence.2-4 The peptide’s capacity to form a triple helix enables CMP hybridization to native or engineered collagens through a thermal annealing process. The CMP replaces a native collagen chain, typically at locations within the triple helix where the collagen is damaged and more susceptible to strand invasion.
Collagen-Based Gene Delivery Systems
Collagen has versatile structural properties, which has allowed collagen-based gene delivery systems to be designed in many forms.1 For instance, solubilized collagen is in a liquid state at cold temperatures but forms a fibrous scaffold at body temperature, permitting pDNA and vectors to be easily incorporated into collagen while in the liquid state. Subsequently, collagen solutions containing the pDNA or vectors can be fashioned into pellets, sponges, films, gels, beads, etc. without heat processing or use of organic solvents; two key sources of vector deactivation common in other delivery approaches. As summarized in Figure 1, each structure has unique benefits.
Collagen Scaffold-Mediated Gene Delivery
Scaffold-mediated delivery has proven to be a promising strategy in overcoming key gene delivery obstacles including off-target effects, immunogenic responses, serum/nuclease instability, and spatiotemporal control. Collagen is a particularly valuable substrate because its diverse structural forms provide different gene delivery profiles, while its similarity to native ECM allows cellular invasion and the corresponding delivery of immobilized genes. Accordingly, over the past two decades, innovative collagen scaffold-based approaches for the delivery of naked pDNA, complexed pDNA, and viral DNA have shown promise in numerous medical applications including bone, wound, cardiac, and optic repair.1
Naked pDNA
The first tissue engineering scaffold to deliver DNA was comprised of collagen and naked pDNA.5 Naked pDNA delivery represents the simplest form of non-viral vector transfection; however, naked pDNA is not efficiently internalized by cells due to its large size and negatively charged phosphate groups. Naked pDNA also suffers from high susceptibility to nuclease degradation and rapid clearance from the body. Despite these drawbacks, collagen-mediated gene delivery was used in a pioneering study to demonstrate that repair cells (e.g., fibroblasts, chondrocytes, and/or osteoblasts) can be genetically reprogrammed in vivo to deliver pDNA encoding osteogenic factors, leading to enhanced bone formation in critically-sized rat femur defects.5 Specifically, collagen sponge-mediated delivery of naked pDNA encoding bone morphogenetic protein-4 (BMP-4) and parathyroid hormone fragment (PHF) synergistically accelerated the functional union of large segmental defects within four or nine weeks when either one plasmid or both plasmids were delivered, respectively. Delivery from collagen matrices has the potential to immobilize pDNA and increase its local concentration and stability in ways that simple injection of pDNA cannot. Furthermore, more controlled delivery of pDNA from collagen matrices has been achieved by regulating matrix degradation rates through implementing different crosslinking strategies and/or altering the degree of plasmid/ matrix integration. Both gene loading efficiency and retention/ release profiles have been expanded using physical crosslinking techniques such as dehydrothermal (DHT) and ultraviolet (UV) treatments, and/or chemical methods including 1-ethyl-3-(3- dimethylaminopropyl)carbodiimide (EDC, Prod. No. E1769) treatments. For instance, EDC and UV crosslinked collagenglycosaminoglycan (GSCG) matrices supplemented with luciferase-encoding genes were demonstrated to induce higher transgene expression in chondrocytes over a four week period relative to non-crosslinked and DHT or UV treated matrices.6 Release from this scaffold was biphasic with an initial burst release period over eight hours followed by a sustained release over the 28-day monitoring period (Figure 2A).
Alternatively, sustained, prolonged pDNA delivery from collagenbased hydrogels has been achieved through the utilization of cationized collagen or gelatin, whose altered charge increases pDNA retention by enhancing electrostatic interactions between the scaffold and the pDNA. Cohen-Sacks et al. demonstrated greatly enhanced pDNA retention in poly-l-lysine-modified versus non-modified collagen hydrogels.7 Moreover, Kushibiki et al. determined that in vivo release and gene expression of pDNA could be tailored by preparing crosslinked hydrogels composed of gelatin with different extents of cationization (Figure 2B).8 Significant in vivo transgene expression was only reported when the aminized percentage of gelatin was 41 mol% or greater. In contrast, prolonged pDNA delivery has also been obtained in atelocollagen pellets without chemical crosslinking. These injectable, dense pDNA-loaded pellets exhibit sustained, prolonged transgene expression in vivo for up to two months compared to three weeks when pDNA was administered alone.9
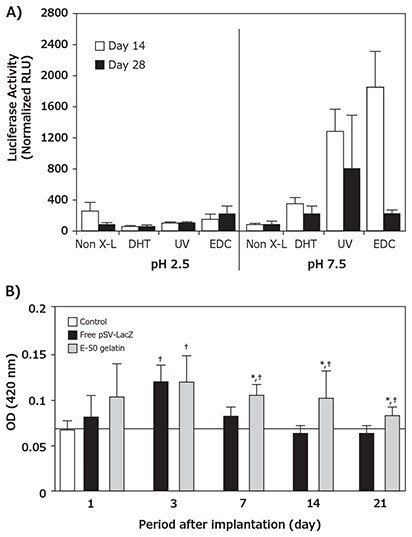
Figure 2:Collagen-mediated naked pDNA delivery A) Different physical and chemical crosslinking techniques promoted significantly different magnitudes and durations of in situ transgene expression within pDNA GSCG matrices encoding luciferase over at 28 day period. B) The implantation of cationized gelatin hydrogels incorporating pSV-lacZ into the femoral muscle of mice promoted sustained and higher transgene expression than free pDNA over a 21 day period. Reprinted with permissions from Mary Ann Liebert (Reference 6), copyright (2002) and Elsevier Ltd. (Reference 8), copyright (2003).
pDNA Complexes
Most non-viral gene delivery approaches rely on the delivery of complexed pDNA nanoparticles created through the electrostatic assembly of pDNA with cationic polymers or lipids. Complexation has the capacity to improve pDNA serum halflives through protection from nucleases, and can also facilitate cellular internalization through increased interactions with anionic cell surfaces. Hence, pDNA complexes typically exhibit higher transgene expression efficiencies relative to naked pDNA.10 Moreover, the use of pDNA complexes offers additional flexibility for regulation of delivery from collagen. Non-specific electrostatic interactions between collagen and gene-loaded carriers, such as lipoplexes and polyplexes, can be tailored through variation of complex charge density and shielding with copolymers to reduce matrix interactions and increase release.10 For instance, Cohen-Sacks et al. demonstrated complexing pDNA with cationic agents like Lipofectamine™ and poly(ethyleneimine) (PEI) enabled prolonged in vivo transgene expression from collagen matrices without the need for linking chemistries or multi-step chemical modification of collagen matrices with cationic polymers.7 Elangovan et al. prepared collagen matrices loaded with PEI polyplexes encoding platelet-derived growth factor (PDGF-B). These scaffolds enhanced new bone formation in calvarial defects by 14-fold and 44-fold when compared to empty defects and scaffolds, respectively, when the defects were evaluated at four weeks post-implantation.11 Vectors have also been engineered with specific affinities for native and modified collagens.2,12 Segura et al. reported the immobilization of PEI polyplexes onto hyaluronic acid-collagen hydrogels through a combination of non-specific adsorption (electrostatic, hydrophobic, and van der Waals interactions) and biotin/NeutrAvidinR binding. These modified scaffolds increased transgene expression 2-fold after a two day incubation in cultured cells.12 Urello et al. demonstrated the integration of PEI polyplexes into collagen scaffolds through CMP/collagen hybridization (Figure 3A), with improvements in DNA loading efficiency and serum-stability, as well as coordination of cell-triggered delivery with extraand intracellular collagen remodeling pathways.2,3 Moreover, variation in CMP display on the polyplexes enabled tailoring of transgene PDGF-B expression within in vitro defect models, which dramatically accelerated wound closure over a 10-day period compared to when collagen containing either low doses of rPDGF-BB or non-modified polyplexes encoding PDGF-B were administered (Figure 3B).
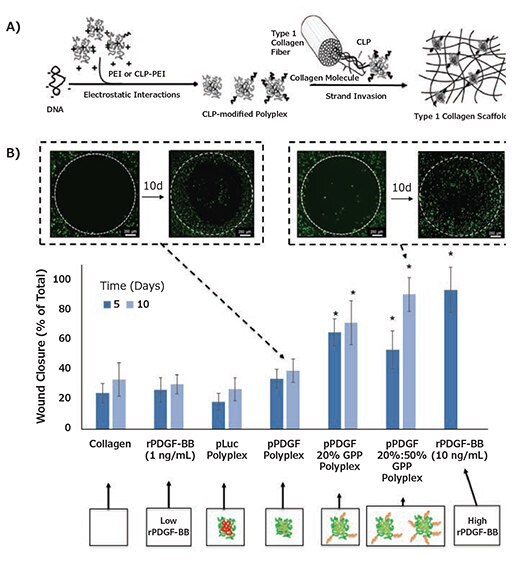
Figure 3. Collagen-mediated pDNA complex delivery A) Schematic of DNA/Collagen modification using CMP hybridization. B) CMP display promoted sustained PDGF-BB expression that resulted in >50% increases in % in vitro wound closure relative to collagen containing either low doses of rPDGF-BB or non-modified polyplexes encoding PDGF-B within 10 days. Reproduced from Reference 2 with permission from The Royal Society of Chemistry and Reference 3 with permissions.
Viral Vectors
Viral gene delivery utilizes the innate ability of viruses to transfect cells with high efficiency. One key mechanism underlying the behavior of many viruses is the ability to bind to the ECM, thereby preventing clearance and increasing cellular availability. Accordingly, the delivery of therapeutic viruses from ECM components, such as collagen, has also been extensively studied.1 Doukas et al. first demonstrated the benefits of biomaterial-mediated viral gene delivery as compared with the direct application of solution formulations of virus or proteinbased therapeutics.13 Specifically, PDGF-B encoding adenovirus formulated in a collagen matrix was shown to significantly enhance healing in rabbit ischemic excisional wounds, whereas repetitive, high dosages of PDGF-BB protein were required to induce neotissue comparable to the single administration of collagen-immobilized AdPDGF-B (Figure 4A). Moreover, the authors demonstrated collagen retained both the vector and transgene products within the wound bed, while the AdPDGF-B vector escaped the wound site when administered in solution and caused PDGF-BB-induced hyperplasia in surrounding tissues. To encourage localized delivery of viral gene carriers, viral vectors have been immobilized onto modified collagens via antibody complexation. Levy et al. first demonstrated successful adenoviral vector delivery based upon anti-adenovirus antibody immobilization within a collagen matrix. Viral vectors were immobilized in type I collagen-avidin gel using a biotinylated, polyclonal antibody specific for the adenovirus hexon. The antibody complexed collagens achieved enhanced, site-specfic, efficient gene transfer in both in vitro cell studies (compared to non-antibody complexed controls, Figure 4B) and in myocardial injection sites in a pig model (compared to direct vector injections).14 Antibody-tethered adenovirus delivery was demonstrated to enhance site specific delivery from collagencoated stents and endovascular microcoils. Alternatively, viruses have been encapsulated into nanocarriers to enhance stability and prevent vector escape from collagen hydrogels. Shin and Shea demonstrated lentivirus-loaded, hydroxyapatite nanoparticles promoted retention in low density collagen hydrogels, were conducive to cell invasion, and promoted overall higher levels of expression in subcutanous mouse implants over a four day monitoring period.15 When the vector is completely immobilized within the biomaterial, vector uptake is reliant on cellular invasion; therefore, the capacity of collagen to facilitate cellular adhesion, proliferation, and migration make it an ideal gene delivery system.
PVA sponges implanted in rates were injected with either 0.15% collagen vehicle or collagen containing AdPDGF-B. Alternatively, PDGF-BB protein was delivered as a single 100 μg dose on day 4 postimplantation, or as 10 μg doses on days 4, 6, and 8. Sponges were removed on day 12 postimplantation and processed to determine the percent granulation fill as well as total protein and DNA contents. Data are presented as means ± SD (n = 4–8).
ap < 0.0001 versus collagen control and p < 0.05 vusus 100 μg PDGF-BB.
bp < 0.03 versus collagen control.
cqod x 3, every other day, three times.
B)
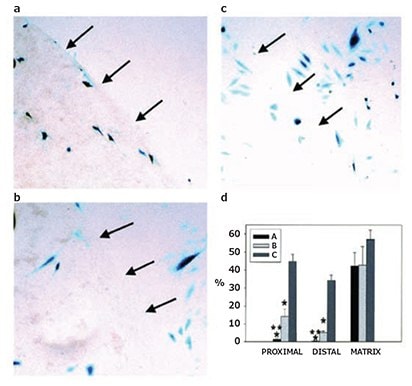
Figure 4.Collagen-mediated viral vector delivery. A) PDGF-B encoding adenovirus (AdPDGF-B) loaded PVA-collagen sponges in a rabbit ischemic wound model facilitated a 53% increase in granulation tissue relative to an empty sponge and repetitive dosages of PDGF-BB was required to achieve a comparable effect. B) Antibody-complexed adenovirus within a collagen- NeutrAvidinR promoted transgene β-galactosidase expression localized to the matrix as indicated in the representative images, where the arrows indicate the edge of the collagen matrix. (a) Complexed-adenovirus; (b) Non-complexed adenovirus; (c) No supplement. (d) The percentage of transfected rat arterial smooth muscle cells expressing β-galactosidase in the matrix, distal (within 100 mm) to the matrix, and proximal (>100 mm) from the matrix. Reprinted with permission from Mary Ann Liebert (Reference 13), copyright (2001), and Macmillan Publishers Ltd: Gene Therapy (Reference 14), copyright (2001).
Collagen Nanocarriers/Microcarriers
Gene-loaded collagen nano-/microcarriers, with collagen as the nano-/microparticle forming agent, represent an alternative to traditional complexation agents and provide a means to form injectable, systemic, and controlled-release systems. Due to the ease of controlling particle size, large surface area, high adsorption capacity, and dispersion ability in water, collagen nanocarriers have been utilized for the sustained release of multiple drugs. Collagen-based nanocarriers have also been shown to prolong circulation time, reduce immunogenic responses, and trigger high levels of cellular uptake. Moreover, collagen-based nanocarriers can be modified with moieties to stimulate targeted delivery and receptor-mediated endocytosis, are able to incorporate multiple plasmids, and can preserve pDNA bioactivity by preventing nuclease degradation. While collagen can form gels without chemical crosslinking, chemical treatments are required to create stable collagen nanoparticles. Wang et al. demonstrated that methylated collagen, DNA carriers were stable at neutral pH and had higher transfection efficiencies in vitro compared to pDNA alone or chemically unstable collagen DNA carriers; however, these findings did not translate in vivo.16
Gelatin has been thoroughly studied as a potential material for colloidal carrier systems. In solution, gelatin undergoes a coilhelix transition followed by aggregation driven by the formation of collagen-like triple helices to enable nanoparticle formation. Additionally, gelatin contains a high number of functional groups that can be used for chemical modification, including crosslinking and functionalization with additional ligands.1 pDNA can be incorporated into gelatin nanocarriers through physical encapsulation, electrostatic interactions, or complexation with surface modifying groups. For instance, negatively-charged type B gelatin was used to physically encapsulate pDNA (at neutral pH) and the resulting hydrogel-like nanocarriers were demonstrated to successfully accumulate within tumors in murine lung and breast cancer models.17 Kommareddy et al. proposed these effects were driven by the enhanced permeability and retention (EPR) effect and the incorporation of a poly(ethylene glycol) (PEG) shielding layer which prolonged systemic circulation time (Figure 5). Alternatively, type A gelatin can electrostatically complex DNA (at pH values below 5) to form coacervate particles, and these particles can then be crosslinked to create physiologically-stable carriers. Crosslinked type A gelatin nanocarriers have exhibited in vitro transfection efficiencies comparable to the commercial transfection agent Lipofectamine.18,19 Moreover, intramuscular injection of these nanocarriers facilitated higher and more sustained transgene expression, in comparison to naked pDNA or LipofectaminepDNA complexes over 21 days.18,19 Cationized gelatin nanocarriers also have been prepared via the modification of gelatin carboxyl groups with amine residues through reaction with cholamine, poly(ethyleneimine), or ethylenediamine. In numerous studies, these particles have been demonstrated to efficiently condense and protect pDNA, promote cellular uptake via interaction with anionic cell membranes, induce endosomal/lysosomal escape through the “proton sponge” effect, and facilitate high levels of transgene expression with low cytotoxicity.19 For additional information, gelatin-based nanocarriers and their applications in drug and gene delivery have been extensively reviewed by Elzoghby.19
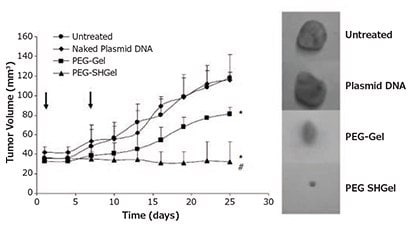
Figure 5: Highlighted nanocarrier example, thiolated and non-thiolated PEGylated type B gelatin nanocarriers loaded with pDNA encoding an anti-angiogenic agent stopped tumor growth for 20 days after intravenous administration in mice bearing orthotopic MDA-MB-435 breast adenocarcinoma xenografts. Reprinted with permission from Macmillan Publishers Ltd: Gene Therapy (Reference 17), copyright (2007).
Conclusions and Outlook
The innate cell adhesive and versatile nature of collagen make it an ideal material for gene delivery applications. Variations in the composition and design of collagen-based carriers and vectors have been used to achieve sustained transgene expression in multiple in vivo models (Figure 6), leading to improvements in bone and tissue repair, tumor targeting, and enhancements in overall gene delivery efficiency, compared with alternative delivery systems. Several key strategies should be considered to further expand the utility of collagen in gene delivery.
Figure 6. Highlighted collagen gene delivery studies in delivery/healing models using a variety of approaches and vectors.
Methods to Improve Intracellular Trafficking
Non-viral delivery strategies must address intracellular trafficking obstacles to obtain transfection comparable to viral vectors. In collagen-based delivery approaches, trafficking has been directed to high efficiency pathways through the inclusion of integrin-targeted peptides, facilitating specific endocytic uptake. Such approaches could be further tailored through the incorporation of nuclear proteins and peptides to enhance nuclear uptake/retention.20
Delivery from Collagen-Based Gels with Better Controlled Properties
The replacement of collagen with CMPs offers great potential in gene delivery, as the CMP sequence and properties are engineered for specific applications. Compared to collagen, CMPs offer additional tailorability, lower antigenicity, and enhanced stability. A diverse array of thermosensitive, self-assembling carriers, and hydrogels have been fabricated utilizing CMPs,4 and the application of these structures in gene delivery has the potential to enable efficient, responsive delivery.2,3
Approaches to Achieve Tailored, Multi-Gene Expression
In applications such as wound healing, multiple therapeutic agents must operate synergistically; this makes sequential and co-delivered gene delivery approaches beneficial. Multiagent delivery has been achieved using layer-by-layer films, encapsulation of therapeutic-loaded nanocarriers, use of additional agents within scaffolds, and engineering different agent/substrate affinities for diverse release profiles.1-3,7 While these strategies have been explored for small molecule drug and protein therapy, few studies have utilized these approaches for gene delivery.
Given the prevalence of collagen within the body and its importance in determining healthy versus diseased states in tissue, collagen-based gene delivery approaches will continue to improve as our knowledge of biological systems and material science progresses.21
Materials
References
如要继续阅读,请登录或创建帐户。
暂无帐户?