Molecular Solar Thermal Energy Storage Systems
Helen Hölzel1, Kasper Moth-Poulsen*1,2,3
1Department of Chemistry and Chemical Engineering, Chalmers University of Technology, Kemigården 4, 412 96 Gothenburg, Sweden., 2The Institute of Materials Science of Barcelona, ICMAB-CSIC, 08193, Bellaterra, Barcelona, Spain, 3Catalan Institution for Research & Advanced Studies, ICREA, Pg. Lluis Companys 23, Barcelona, Spain
Material Matters™, 2022, 17.3 Material Matters™ Publications
Introduction
With worldwide population growth, the global energy demand has drastically increased and will rise by an average of 1.3% annually until 2040.1 Currently, this challenge is not merely addressed by conventional energy sources such as oil, coal, gas, or nuclear power, but also by renewable energy sources such as wind and solar energy. The demand for renewable energy sources has been increasing even during the economic crisis due to lockdowns caused by the global pandemic, Covid-19.2,3 The renewable energy share, among the total energy mix, increased to 5.7% in 2020, outnumbering nuclear energy at 4.3%.3 The growth for wind and solar energy was 238 GW in 2020, which is 50% more than any single year in history. The sun, being most abundant among renewable energy sources, delivers around 235 Wm-2 on average.4 Typically, energy from the sun is used directly for heating or electric power production; however, as renewable energy continues to grow as part of the energy mix, efficient energy storage becomes a growing challenge. A promising approach for solar energy harvesting and storage is the concept of molecular solar thermal energy storage (MOST) systems also known as solar thermal fuels (STF). Solar energy is used to drive the chemical reaction of a molecule, usually referred to as a molecular photoswitch, leading to an energy-rich metastable isomer, which stores the energy. The energy can later be released on demand, controlled thermally, catalytically, or through irradiation with selected wavelengths of light. In this article, we introduce the requirements for a MOST system, the structures of different photoswitches, their general charging and discharging mechanisms, highlight the accessibility of the material by synthetic production, and describe possible uses of the stored energy.
Molecular Solar Thermal Energy Storage (MOST) Systems
In general, MOST systems should feature at least four functional principles as illustrated in Figure 1A. A MOST system is based on a photochemical reaction such as isomerization, dimerization, or rearrangements. During the photochemical reaction, photon energy is converted to chemical energy by converting the parent molecule, A to a high-energy meta-stable photoisomer, B (Figure 1). B should have a high-energy storage density compared to A, and depending on the application, should feature a suitable storage half-life (t1/2). The back-reaction process should result in a release of energy as heat and can be activated by either thermal activation, a catalytic system (catalyst or electrochemistry), or light. The last but most important principle is the stability and availability of the system. This includes a simple way of molecule preparation, which requires economic feasibility regarding applications. Further, the molecule should be stable for longer periods, and withstand several cycles of operation. To meet all these principles, molecular design is the most crucial point.
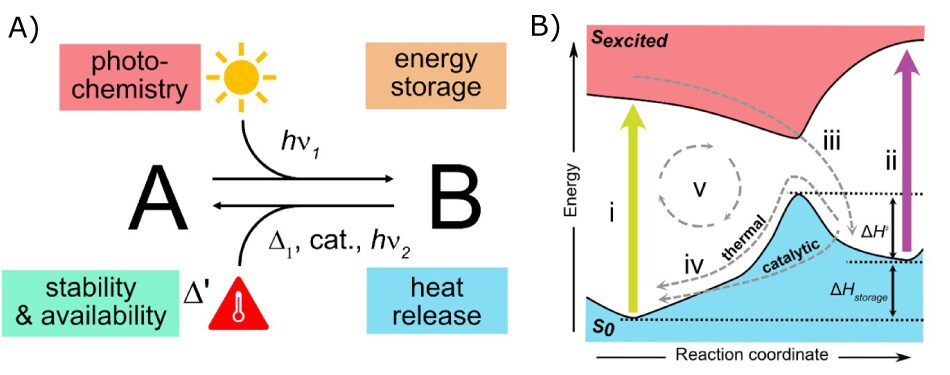
Figure 1.A) Schematic depiction of features of a MOST system and B) the MOST operation cycle, i) absorption from parent molecule to an excited state, ii) absorption from the meta-stable state, iii) photoconversion, iv) thermal or catalytic back-conversion, v) cyclability. Reproduced from reference 5, copyright 2022 Elsevier B.V.
Before design and synthesis come into play, it is necessary to understand the energy landscape and steps of the energy storage process in more detail, to extract the most ideal concept fitting the requirements to create efficient systems.5–7 The process consists of four main steps and a few side processes (Figure 1B). Exposure to light should excite molecule A from its ground state (S0) to its excited state (Sexcited) via photon absorption (i). To use the photons provided from solar irradiation, the system should absorb light between 300–800 nm,8 as 50% of the incoming photons are within this range.5 Through photoconversion (iii) the excited molecule ends up in a meta-stable high energy photoisomer B. This process strongly depends on the photoisomerization quantum yield φiso. Preferably, the photoisomerization should happen via one photon with a quantum yield close to 1. The molecule should then remain in this state, for longer periods indicated by the half-life time (t1/2). Depending on the application, t1/2 should be long enough to store the energy for days, months, or years at room temperature.8 However, t1/2 depends on the rate of the back-reaction (iv) and is thus intimately related to the thermal back-reaction barrier ΔH‡. As the meta-stable isomer should store energy, the ΔHstorage, which is the energy difference between the two isomers, should be as high as possible. To release the energy, the back-conversion (iv) can be triggered either catalytically, thermally, electrochemically, or for some compounds also photochemically. Especially the latter trigger might display a competing absorption process (ii) and should be avoided or suppressed. In the best case, the spectral overlap should be small. The trigger should be as efficient as possible to release the energy when required. Considering this is a circular process, the final, important factor is the cyclability (v) of charging and discharging of the MOST material. Within this cycle, there should be no degradation (or less degradation) and no material fatigue should be observed. Real-life applications should apply sustainability practices, meaning the material should be harmless for the environment and humans. These requirements are very specific and difficult to address in a single molecular system, creating a challenge to find the “ideal system” to apply in real-life applications. Nevertheless, the search steadily reveals important molecular features and progress in performance in all aspects previously mentioned. In the following sections, we will introduce different molecular systems that are being studied and how molecular design has been used to improve their function.
Photoswitches molecules as MOST Materials
Through the years several different molecular systems have been investigated and suggested as MOST candidates.5,6 Three main molecular systems are gaining increasing interest: the norbornadiene-quadricyclane (NBD/QC), the E/Z-azobenzene (E/Z-AZO), and the dihydroazulene/vinylheptafulvene (DHA/VHF) isomers (Figure 2).
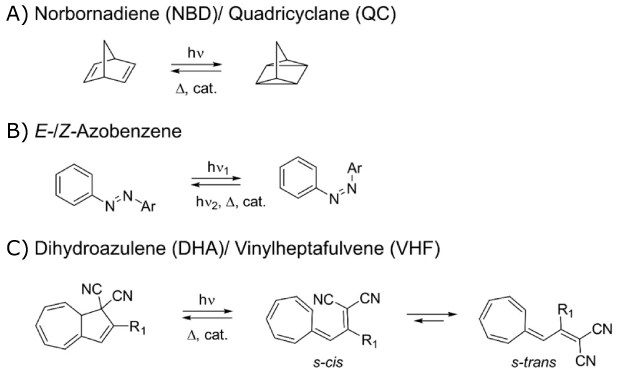
Figure 2.Examples of currently investigated MOST systems and conversion processes. A) norbornadiene NBD/ quadricyclane QC couple, B) E-/Z-azobenzene couple, C) dihydroazulene DHA/ vinylheptafulvene VHF couple.
However, while the overall goals for the use of those systems as MOST are similar, there are some differences in the charging and discharging mechanisms as well as molecular design principles for each compound class. For the NBD/QC system the molecule is excited through either direct irradiation or via the use of a photosensitizer. The chemical reaction that occurs after excitation is a [2+2] cycloaddition (Figure 2A). Unsubstituted NBD itself only absorbs in the UV region, therefore different design strategies have been used to optimize the system for solar absorption.9 The quadricyclane molecule has a high internal strain and can store around 0.1 MJ mol-1.10 For some optimized compounds storage energy densities of up to 1 MJ kg-1 are reported.7,11 The azobenzene system can be switched from its E/trans-isomer to its Z/cis-isomer via irradiation at the respective wavelength (Figure 2B).6 However, the amount of stored energy of the parent Z-azobenzene (0.041 MJ mol-1) is around half the value of QC,12 and full conversion is challenging to achieve due to the photochemical equilibrium. The Z-isomer converts back to the E-isomer via light irradiation in the visible range. This competition can be mitigated using a bandpass filter for device applications13 or by molecular engineering.14 The DHA molecule can be converted to VHF through a photoinduced ring-opening reaction. Initially, the s-cis form is formed which then changes into the more stable s-trans conformer (Figure 2C).15 The VHF usually possesses red-shifted absorption compared to DHA, similar to azobenzene, but is photochemically inactive, and thus no competing back-conversion of the visible spectral range occurs. To date, most parent molecules cannot store energy efficiently; therefore, structural, and molecular design strategies are employed to modify and optimize the properties.
Molecular Design Strategies
Addition of substituents to red-shift the absorption profile of the parent molecule A is an important objective for storage of a large fraction of the solar energy spectrum. Herein, we exemplify some strategies for the NBD molecule.9 Attaching corresponding functionalities and substituents to the parent molecules using a donor-acceptor pair, lowers the HOMO-LUMO gap of the system. Hereby two design approaches can be used. One approach is the introduction of donor and acceptor groups at the two different double bonds of the NBD system (Figure 3A).16 These types of push-pull systems, NBD 1, rely on the principle of homoconjugation which promotes communication and charge transfer through space. However, this may be accompanied by an increase in molecular weight which reduces the energy storage density. Another approach is a push-pull architecture on one double bond of the molecule (Figure 3B, for example, NBD 2).11 This results in a better match with the solar spectrum via bathochromic shifting of the absorption profile but may lead to reduced energy storage time t1/2.
Dimeric donor-acceptor type systems, NBD 3, can lead to even further red shifting of the absorption band and provide enhanced charge transfer through the bridge (Figure 3C).17 Accompanied by the general design of multi-photoisomeric architectures, which feature two or more photoswitchable units. Each moiety can exhibit different thermal back-conversion barriers and thus lead to several switching events in one molecule so that the overall energy storage density increases even though the molecular weight also increases.18 Therefore, one of the most promising approaches to date, utilizes two concepts: the extension of the conjugation within the system and the introduction of electron-donating and electron-accepting groups. Aryl and acetylene units attached to the molecular core typically led to a better match with the solar spectrum and show enhanced p-conjugation. However, this increases the molecular weight drastically, especially in the case of aryl units.19 To counter this, one of the substituents can be replaced by a low molecular weight functionality with electron-withdrawing properties.20 This could result in very promising candidates such as NBD 4 (Figure 3D) which not only lead to bathochromic shifting of the absorption but also a higher energy density, as well as improved and persistent stability, withstanding many cycles of charging and discharging.21 Unfortunately, in this case, a lowered t1/2 was observed.20 Extension of storage times for NBDs was observed by the addition of bulky substituents to the carbon bridge or the introduction of ortho-aryl substituents (compare NBD 5 and NBD 6, Figure 3E). Both approaches lead to steric repulsion effects directly impacting the back-reaction barrier.11,22
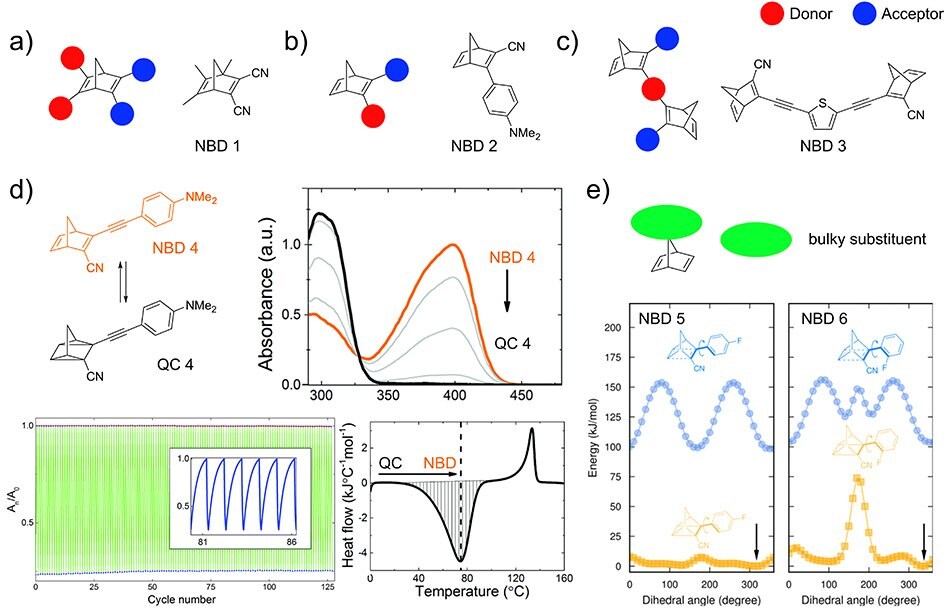
Figure 3.A–C) Different NBD donor-acceptor motifs with examples, D) example for a low-molecular weight NBD with red-shifted absorption and full conversion, high cyclability, and good heat release, E) strategy to improve half-life by introduction of bulky substituents or change in the dihedral angle using ortho-substituents. Figures reproduced from references 11, 20 and 21, copyright 2016 Wiley-VCH Verlag GmbH&Co. KGaA, 2018 Wiley-VCH Verlag GmbH&Co. KGaA, and The Royal Society of Chemistry 2017.
Similar Design strategies are applied for other MOST systems, e.g. azobenzenes and DHA (Figures 4A and 4C). In a recent study, substituents on the azobenzene units had stark effects on their properties (storage times, absorption profiles, isomerization quantum yield, etc.), e.g. donor-acceptor motifs or introduction of ortho-substituents, especially fluorinated azobenzenes (Figure 4A and 4B).6 Leading to exceptional extended half-life times caused by less electron density in the azo-bridge, thus stabilizing the Z-form.6 Further half-life and red-shifting of absorption can be influenced by introducing various amino-functionalities with different p-donation properties; hence stronger donors lead to more visible range spectral overlap while weaker donors ensure longer storage times (Figure 4B).23 Replacement of the phenyl-substituents in azobenzenes by using heteroaryls (Figure 4B), such as triazoles and pyrazoles have an impressive effect on the conversion, revealing complete conversion from Z to E in most of the cases.6,14,24 In the case of bis-pyrazole systems, less steric hindrance within the molecule and the position of substitution of the heteroaryl moiety, effects the thermal back-reaction and thus the half-life of the system, due to different stabilization via intramolecular interactions.14 Azobenzenes have also been investigated as phase-change materials for MOST applications.25 DHA molecules are also influenced by substituents (Figure 4C), especially with the introduction of donor-acceptor units at the C2, C3, or C7 positions, for example, with functionalities attached to phenyl groups.15,26,27 Hereby, longer half-lives and red-shifting of absorption were achieved, especially when using cyano-based substituents at C7.28 Variations from one cyano group at C1 using a hydrogen atom or methyl group influence the back-reactions barrier,29 while computations reveal that annellation of a benzene unit at the C2–C3 will increase the energy density.30
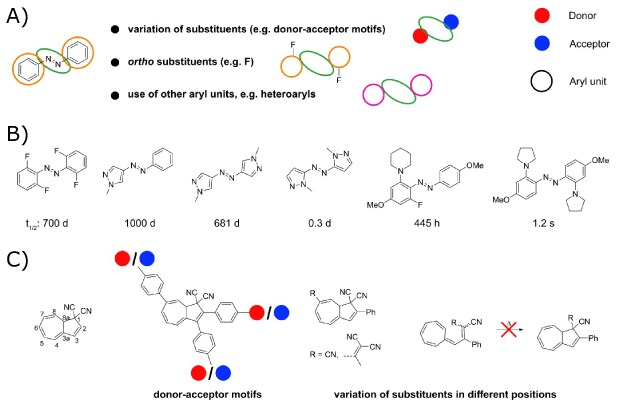
Figure 4.A) Examples for variation of azobenzene, B) concrete examples and influence on the half-life, C) numbered DHA molecule and examples for variation.
Synthesis and Preparation
The preparation of MOST materials is strongly dependent on the molecular system and the accompanying structural composition. In the case of NBDs, synthesis occurs primarily using two approaches: palladium-catalyzed cross-coupling reactions, or Diels-Alder reactions (Figure 5). The first synthesis pathway starts from the commercially available 2,5-norbornadiene (Cat. No. 8.20918) 1 (Bicyclo[2.2.1]hepta-2,5-diene, Cat. No. B33803) which reacts with brominating agents, like 1,2-dibromoethane 2, (Cat. No. 240656),31,32 or by using a lithiation followed by quenching with electrophiles, such as p-toluenesulfonyl halide, to form a halogenated species 2.33 These molecules can then undergo cross-coupling reactions such as Sonogashira or Suzuki reactions using palladium catalysts (Figure 5A). With this approach, several 2,3-substituted NBDs 3 were synthesized.11,20
The second approach includes the use of cyclopentadiene (CP) 6 and substituted acetylene 7 in a [4+2] cycloaddition reaction, here a Diels-Alder reaction (Figure 5B). However, this electrocyclic reaction requires specific electronic properties of both reactants. Usually, it involves 4p-electrons of the diene and 2p-electrons of the dienophile. To match the electronic requirements, the HOMO of the diene and the LUMO of the dienophile need to overlap. This is even more favored when the dienophile carries an electron-withdrawing group (EWG, e.g. COR, COOR, CN) which facilitates the reaction by lowering the LUMO energy. Using the EWG group on the acetylene, the reaction with the reactive and electron-rich diene can be carried out easily, to result in NBDs 8. Also, depending on the starting material this reaction can be performed neat, without involving any solvent. As shown previously, this synthesis route can be efficient for the preparation of various types of NBDs.11 However, this approach also gives rise to challenges since the cyclopentadiene is unstable and usually forms an equilibrium with its dimer, dicyclopentadiene 9 (DCPD, Cat. Nos. 8.03038 or 454338). CP can be regained from DCPD 9 via a retro Diels-Alder reaction, through thermal cracking (Figure 5C). For NBDs requiring a higher temperature for synthesis, both reactions can be carried out in a combined fashion.
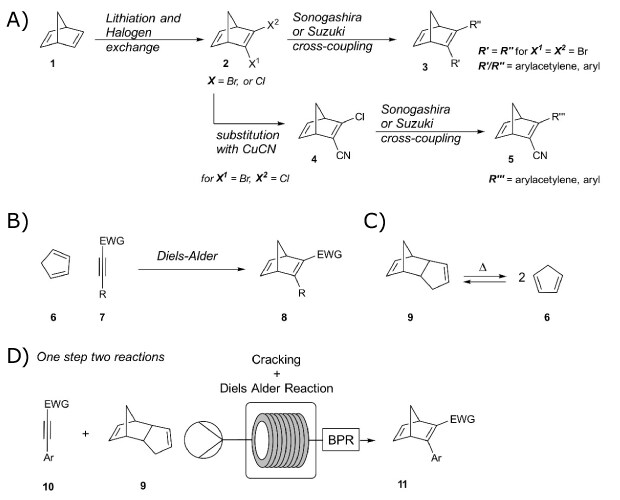
Figure 5.Synthetic approaches of norbornadienes as MOST material. A) Lithiation and Halogen exchange starting from norbornadiene, option for replacement of bromine with nitrile via substitution, and with subsequent cross-coupling reactions. B) Diels-Alder reaction between cyclopentadiene and activated acetylene. C) Dicyclopentadiene cracking to cyclopentadiene under thermal conditions. D) Recently developed combined cracking and Diels-Alder flow method to norbornadienes.
Most synthetic approaches were carried out in batches, which becomes impractical when upscaling is required. Therefore, another method gaining attention comes into play: flow chemistry. Recently, a continuous flow method that combines both the Diels-Alder and Cracking in one step was developed using a tubular flow reactor allowing for the preparation of larger quantities (Figure 5D).34 Herein, a commercially available dienophile, ethyl phenylpropiolate (Cat. No. E45309), CP 6, and DCPD 9, were used for screening and optimization of the reaction in a 5 mL stainless steel coil reactor. Optimized conditions were then applied to various substituted acetylenes 10 to synthesize differently functionalized NBDs 11. The method shows that it is possible to upscale and produce around 100 g of NBD in a few hours. Applications need larger amounts of material, which makes this method very valuable.
Azobenzene systems can be synthesized via various approaches.35 The most common are Azo coupling and the Mills reaction. Azo coupling uses a diazonium salt as electrophile prepared from a primary amine with NaNO2 (Cat. No. 901903 or 563218) in acidic media, and an electron-rich partner, such as arene derivatives decorated with electron-donating groups, while the Mills reaction includes a nitroso-derivative which is typically reacted with aniline in glacial acetic acid. DHA molecules have been prepared mainly through three pathways.36 The first approach follows a [8+2] cycloaddition between 8-methoxyheptafulvene and dicyanoethylenes, the latter prepared from malononitrile (Cat. No. M1407) and carbonyl compounds. The second method directly starts from tropylium tetrafluoroborate (Cat. No. 164623) which either first reacts with a dicyanoethylene compound or a carbonyl derivative and then with malononitrile. This subsequently leads to the formation of a VHF molecule that reacts under heat to the corresponding DHA compound. The third route which primarily results in a substituted 7-membered ring relies on the utilization of tropone derivatives which forms the VHF, form but via condensation with dicyanoethylenes, which can then be converted to the DHA form using heat.
Energy Release in MOST Systems
MOST systems are unique energy systems in the sense that they offer energy capture, storage, and release in a single, emission-free system. The challenge is to design devices that make use of these attractive properties while mitigating challenges with low energy storage efficiency and energy density. In one implementation, a liquid MOST system is first pumped through a solar collector, and later, a heat release device containing a fixed bed catalytic system is used to trigger the heat release (Figure 6A left). In this way, chemical energy from the QC to NBD conversion was used to increase the temperature in the device from ambient temperature to 85°C (Figure 6A right).37 This heat release can also be used to heat water in a hybrid device (Figure 6B).21 Electrochemistry can also be used to trigger heat release. In this way, the initial oxidation of QC leads to the conversion of several molecules by a local radical mechanism (Figure 6C).38 Research shows this reaction works reversibly both in solution and in solid-state and offers an attractive way of controlling energy release.
Other systems feature hybrid combinations of MOST and phase change materials to allow for continuous thermal heat storage and release over extended periods (Figure 6D).39 The co-location of the energy capture storage and release together with the semitransparency of most systems allows for unconventional applications, such as the possible integration of the MOST system into functional windows40,41 where the MOST system is intended to regulate the daily variation of solar influx, where in many cases, strong solar influx at noon leads to strong heating, whereas energy loss through windows at night need heating (Figure 6E). A functional MOST system may in the future be used in this way to control indoor climates.
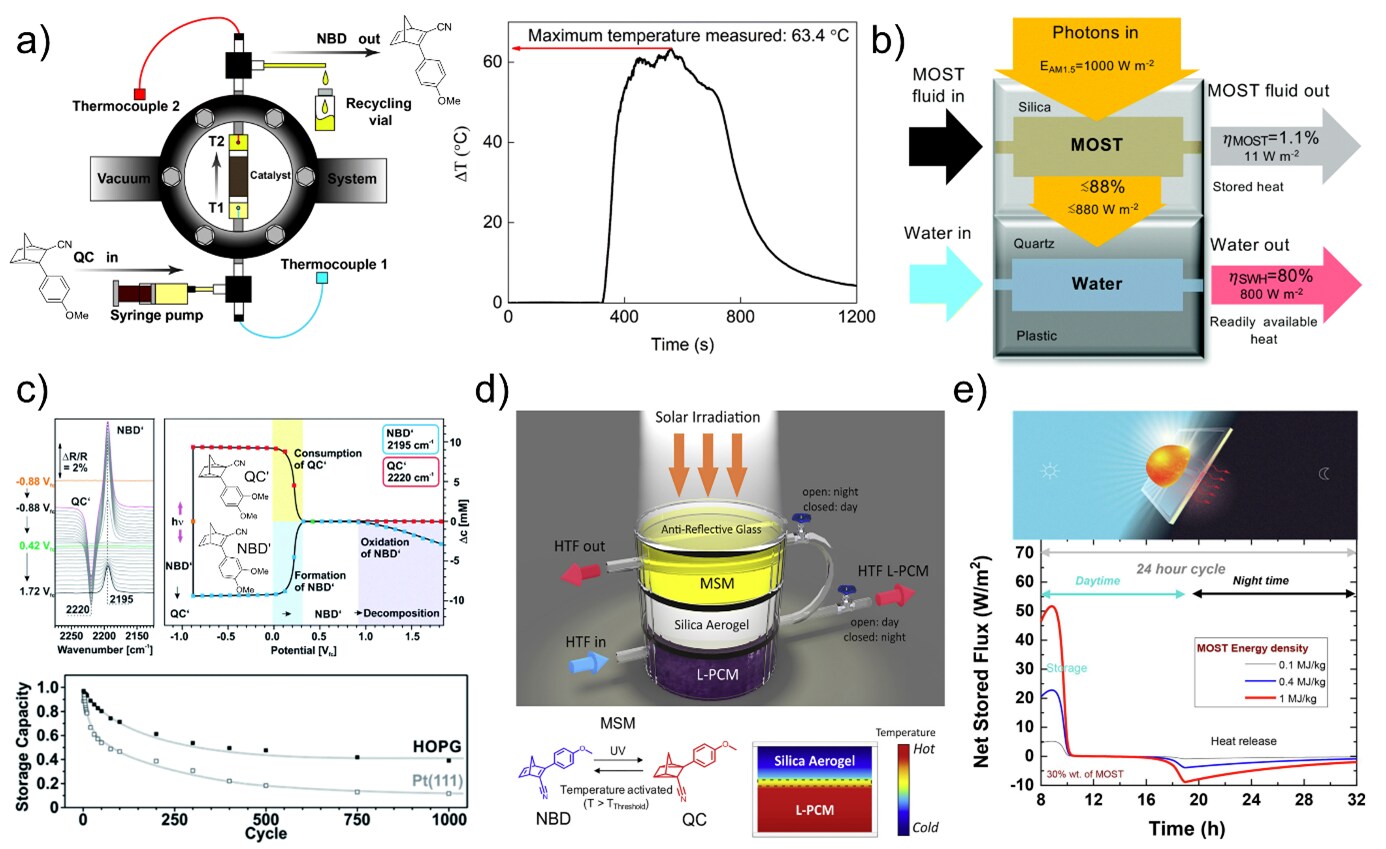
Figure 6.Examples of energy release and devices for conversion. A) Illustration of a heat release device and corresponding device plot. B) Illustration of a hybrid device used to heat water. C) Plots of energy conversion of several molecules by a local radical mechanism. D)Illustration of hybrid combinations of MOST and phase change materials allowing continuous thermal heat storage and release over time. E) Plot of a 24H cycle using MOST in a functional window. Figures reproduced from references 21, 37-39, 41, copyright The Royal Society of Chemistry 2019, The Royal Society of Chemistry 2017, The Royal Society of Chemistry 2020, 2019 Elsevier Inc., and 2022 by Elsevier Ltd.
Summary
MOST systems were first proposed for energy storage more than 100 years ago.42 Recently, increased efforts have been carried out to improve the functionality of molecular photoswitches for solar energy storage. Herein we have introduced the features of MOST systems and presented design principles that have been used to increase energy storage density, efficiency, and availability, we have highlighted key examples of synthesis from literature. With increasing awareness of challenges with traditional energy production and geographic uneven distribution of fossil fuels, we hope that new, emission-free solar energy systems can be developed since the sun shines on all areas of the planet.
Related Products
References
To continue reading please sign in or create an account.
Don't Have An Account?