Progress in Spintronic Materials
Soubantika Palchoudhury1, Karthik Ramasamy2, Arunava Gupta3
1Civil and Chemical Engineering, University of Tennessee at Chattanooga, Chattanooga, Tennessee 37403, USA, 2Center for Integrated Nanotechnologies, Los Alamos National Laboratory, Albuquerque, New Mexico 87185, USA, 3Center for Materials for Information Technology, The University of Alabama, Tuscaloosa, Alabama 35487, USA
Introduction
Spin-based electronic (spintronic) devices offer significant improvement to the limits of conventional charge-based memory and logic devices which suffer from high power usage, leakage current, performance saturation, and device complexity.1,2 Spintronics, as first recognized by Mott in 1936, is the interlinking between the spin and charge degrees of freedom of a material.3 In particular, magnetic materials are highly promising for use in spintronic devices because their electron spin orientation can be readily manipulated through external magnetic fields. The difference in mobility and the population of spin up and spin down electrons in magnetic materials induce a net flow of spin-polarized current. Due to the intrinsic hysteresis properties of ferromagnetic materials, a spintronic device can ideally “remember” the set state for an indefinite time. Consequently, these non-volatile spin-based materials allow significant increase in device density and energy savings while also offering new functionalities.4
Current spintronic applications focus primarily on magnetic storage and sensing, using giant magnetoresistance (GMR) and tunneling magnetoresistance (TMR) effects (Figure 1). GMR is observed in thin films with alternate ferromagnetic and non-magnetic metallic spacer layers. The enhanced resistance observed when ferromagnetic layers are magnetically aligned anti-parallel to each other, rather than aligned parallel, forms the basis of GMR. A TMR device, in its simplest form, consists of two ferromagnetic metallic electrodes on either side of a very thin non-magnetic insulator layer. Electrons are transmitted from one ferromagnetic electrode to the other through quantum mechanical tunneling across the insulating barrier. Similar to GMR, the resulting tunneling conductance depends on relative alignment of the ferromagnetic layers and is the underlying principle behind TMR. The GMR and TMR effects have been exploited and widely used in hard-drive read heads and, more recently, in magnetic random-access memory (MRAM) devices.
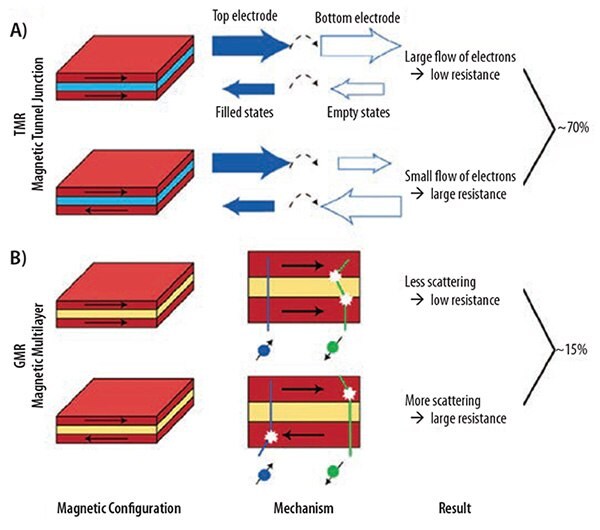
Figure 1.Spintronic devices. A) TMR and B) GMR.1
The control of magnetism and spin has far-reaching potential outside its conventional applications. This is confirmed by many rapidly advancing spintronic subtopics such as current-induced torque, spin Hall effect, spin caloritronics, silicon spintronics, and the spintronics of graphene and topological insulators.5 Current-induced magnetization dynamics is based on the principle that a change in the direction of the electron spin from interaction with a thin ferromagnetic layer induces in-plane or perpendicular spin-transfer torque on the ferromagnet. This manipulation of magnetization has become a key tool for the exploration of nextgeneration devices such as logic-based spin-transistors, miniaturized MRAMs, and high-density data storage. The spin Hall effect, originating from the spin-orbit coupling interaction that orients the electrons perpendicular to the current in a spin-dependent way has emerged as a valuable tool to envisage spin-current measurement technology like fieldeffect transistors (FETs). Spin caloritronics is based on the spin Seebeck effect, but a complete understanding is still lacking. It is a remarkable concept for generating spin current and can potentially be exploited to enhance the efficiency of heat engines. Silicon spintronics is an attempt to revolutionize information technology through the integration of nonvolatile ferromagnetic memory and band-gap engineered semiconductor logic computing.6 In addition, recent experimental realization of graphene and topological insulator surface states opens new routes for spin generators (topological insulators) and spin conductors (graphene). Graphene and topological insulators both share the linear Dirac spectrum, but represent two polar opposites of paramagnetic conductor spintronics. Topological insulators are also a key component in fault-tolerant quantum computation as they can be integrated with superconductors to generate Majorana fermions.
The fast-paced development and tremendous potential of spintronic devices are facilitated through the combination of fundamental solid-state physics and materials research. A central goal of spintronics has been to discover new materials with prominent novel spin-based mechanisms.7 For large-scale practical realization of spintronic devices, they must outperform the switching time, energy per switching event, and output signal of current semiconductor devices. Enhanced efficiency for generation, transport, and detection of spin-polarized carriers is essential to meet this goal. Consequently, the development of novel materials with high spin-polarized carriers, efficient transport, and enhanced room temperature GMR and TMR effects is critical for the exploration of new directions in spintronics. This review summarizes the emerging classes of spintronic materials and the key properties targeted in developing these materials so far.
Emerging Materials for Spintronic Devices
Heusler Alloys
A class of intermetallic solids, referred to as Heusler compounds, represents a remarkable class of materials with more than 1,500 members, with new compounds synthesized on a regular basis. While Heuslers (an eponym based upon the original discoverer) have been known for a long time, it is only in recent years that a wide range of their extraordinary functionalities, including ferri- and ferromagnets, multiferroic shape memory alloys, thermoelectrics, and topological insulators, have attracted significant attention for spintronic, energy, and magnetocaloric applications.8 The two primary classes of intermetallics are the Full-Heusler with X2YZ composition (X, Y: transition metal atoms and Z: semiconductor or non-magnetic metal) having an L21 crystal structure, and the Half- Heusler with XYZ composition and C1b structure. Heusler alloys ofboth compositions are attractive candidates for spintronics applications and have been extensively studied over the past decade. In particular, room temperature (RT) materials with essentially 100% spin polarization (referred to as half-metallic) and high magnetocrystalline anisotropy areideal for next-generation spintronic devices. Crystalline disorders likeatomic displacement, misfit dislocation, and symmetry breaks near the surface of the film are primary obstacles to meeting this target in the Heusler compounds.
Two major criteria can be identified to assess the RT half-metallicity and, consequently, the potential of Heusler alloys as spintronic materials. First, the ability of the Heusler alloy to show over 100% GMR ratio at RT and second the demonstration of >1,000% TMR. In 2011, a 74.8% GMR ratio was reported with a Co2Fe0.4Mn0.6Si/Ag/Co2Fe0.4Mn0.6Si junction, which is a significant improvement over previously obtained values.3 With this GMR stack and other recent developments, the Heusler alloys are frontrunner contenders to satisfy the 2 Tbit/in2 areal density in hard disk drives. Based on recent technology roadmapping, these materials are expected to achieve the target 100% GMR ratio in the coming years. In terms of TMR ratio, magnetic tunnel junctions (MTJ) with MgO spacers exhibit the highest TMR effect, resulting from intrinsic spin-filtering behavior of MgO-junctions as opposed to simple tunneling. For example, a tri-layer stack of CoFeB/MgO/CoFeB showed a TMR of 604% at RT. While this is the largest TMR so far, this material’s low thermal stability and scalability may limit its potential.9 An additional advantage of a number of Heusler alloy films with high spin polarization is their low damping constant. In 2005, the Co2MnSi/Al-O MTJ was shown to exhibit a large TMR of 70% at low temperatures, but the TMR degraded rapidly at RT. Significantly larger TMR ratios were observed in Heusler alloy films with an MgO barrier due to the coherent tunneling effect in MgO. In particular, a Co2FeAl0.5Si0.5/MgO MTJ showed a RT TMR of 386% (832% at 9 K), the highest for Heusler alloys so far. However, an even higher RT TMR ratio is required for the Heusler alloy films to replace CoFeB-based MTJs as read sensors. Based on current research, Heusler alloy films are expected to meet 1,000% TMR or RT halfmetallicity by 2024 (Figure 2).
Heusler alloys and, in particular, cobalt-based Heusler alloy films are expected to be integrated into GMR read head technology by 2018. In addition, these films can be used in combination with nonmagnetic or antiferromagnetic Heusler alloy films to form novel Heusler heterostructures for next-generation MRAMs. The integration of antiferromagnetic Heusler alloys has the potential to improve film quality by inhibiting crystalline disorder at interfaces. Nayak et al. reported that the Heusler alloy Mn2PtGa shows a large exchange bias effect due to antiferromagnetic coupling at low temperatures.10 The cubic Half-Heusler CuMnSb is also known to be antiferromagnetic below 50 K. However, a related tetragonal phase of composition CuMnAs has recently been epitaxially stabilized in thin film form that exhibits antiferromagnetic behavior at room temperature.11 These breakthroughs are a significant step toward realization of exchange-biased all-Heusler alloy GMR and TMR heterostructures. Figure 2 shows the anticipated roadmap of Heusler alloys towards RT half-metallicity and spintronic applications. Recent research has also focused on Heusler nanoparticles, which are expected to exhibit size and shape-dependent spintronic properties.12 Interest in Heusler nanoparticles was primarily catalyzed by the significant influence of size and interfaces on spintronic devices and the observation of granular-GMR. But the field is still in its infancy with efforts directed at shape-controlled synthesis of nanoparticles like Co2FeAl, Co2FeGa, or Co2NiGa.
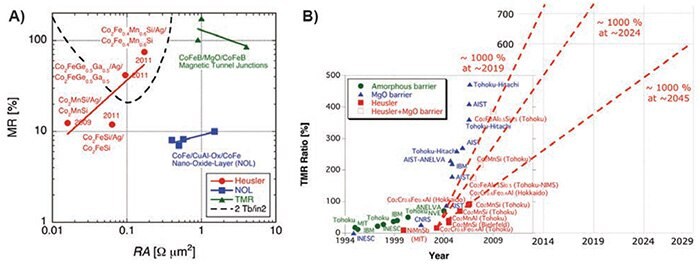
Figure 2.Recent progress of Heusler alloys in spintronics. A) Requirement of Tb/in2 HDD read head and recent major results, and B) developments in TMR ratios.3
Magnetic Oxide Materials
Transition metal oxides exhibit a wide range of tunable magnetic and electrical properties that are very attractive for spintronics. For example, the doped perovskite system La1-xSrxMnO3 (LSMO, x=0.3–0.4) is both metallic and ferromagnetic, with near-unity spin polarization. In combination with the perovskite material SrTiO3 as a tunnel barrier, LSMO has exhibited a TMR ratio as high as 1,850%.13 But such a high TMR value is observable only at low temperatures. In order to achieve high TMR at RT, magnetic double perovskites that have a higher Curie temperature have been explored. In double perovskites, the formula unit is doubled by the presence of two B cations, i.e., A2B´B´´O6, ideally with a completely ordered occupation of octahedral sites. Bulk studies identified the compounds Sr2FeMoO6 and Sr2CrReO6 as showing magnetic ordering above RT with promising half-metallic characteristics.14 But high-quality tunnel junctions based on films of these complex oxides have been challenging to fabricate, primarily because of the narrow process window for high-quality film growth and the propensity for B site disorder that degrades the magnetic properties. Another metallic ferromagnetic oxide of interest is CrO2 which has been widely used in the past as a particulate medium in magnetic tapes. It has a tetragonal rutile structure with a moment of 2.0 μB/f.u. and a Curie temperature of approximately 400 K. CrO2 has also been experimentally confirmed to be half-metallic.15 Novel heterostructures of CrO2 combined with other members of the rutile family, such as TiO2, VO2, RuO2, and SnO2, are expected to show exciting spintronic properties. For example, band structure calculations suggest that heterostructures of CrO2 with metallic or semiconducting rutile oxide spacer layers can potentially exhibit very high magnetoresistance. However, there has been limited work reported thus far on CrO2-based devices, primarily because of the difficulties related to thin film synthesis of this metastable phase. The surface of CrO2 tends to readily reduce into the more stable phase Cr2O3, which is antiferromagnetic. Nevertheless, there has been some success in fabricating rutile heterostructures of CrO2, and ongoing investigations indicate the possibility of realizing the full potential of this unique material for spintronics. Spinel phase iron oxide (Fe3O4), which is the oldest known magnetic material, is another widely investigated material system for the spintronics applications. It has a high Curie temperature of ~850 K and is predicted to exhibit high spin polarization (~80%). Over 500% magnetoresistance ratio has been observed for Fe3O4 through nano-contact measurements.16
While ferromagnetic Fe3O4 displays hopping conduction resulting in poor metallic behavior at RT, other ferrites of the spinel family, e.g., NiFe2O4 and CoFe2O4, are insulating. These ferrites are widely used for high frequency applications, and thin films of these materials have recently attracted interest in spintronics research for spin filtering and spin caloritronics. By using a magnetic insulator as a tunnel barrier, one can ‘filter’ out spins of one orientation and achieve 100% polarization even without half metals. Effective spin filtering has been demonstrated at low temperatures using ferromagnetic semiconducting EuO.17 More recently, several insulating spinel ferrites have been shown to act as RT spin filters but, thus far, the TMR for these have been quite low. Another rapidly expanding research field of magnetic insulator-based spintronics involves efficient transport of spin current and magnons without any charge transfer.5 This requires magnetic materials with very low damping. The insulating ferrimagnet yttrium iron garnet (YIG, Y3Fe5O12), which is widely used in microwave and magneto-optical applications, is presently the preferred material for most studies of spin transport and dynamics since it exhibits exceptionally low damping even in thin films. Nevertheless, there is a great need for developing new insulating and semiconducting magnetic oxides for efficient spin generation and transport for room temperature device operation.
Multiferroic Spintronic Materials
Multiferroics are materials in which at least two of the ferroelectric, ferro/antiferro magnetic, and ferroelastic phases co-exist. The technological appeal of multiferroic materials is huge, particularly for designing multifunctional hybrid spintronic devices, as these materials have the potential to control magnetism with electric fields (Figure 3).18 Hybrid nanoscale devices based on multiferroics are expected to drastically reduce dissipation losses and have a potential market value worth billions of US dollars. Classic multiferroic materials like antiferromagnetic Cr2O3, boracites, rare-earth ferrites, and manganese perovskites have been known since the 1950s, but these materials have Neel or Curie temperatures below RT or are challenging to grow as thin films. These limitations and a lack of knowledge about the guiding mechanisms behind the multiferroic effect brought the field to a stand-still after initially showing significant promise. Only recently, groundbreaking discoveries in the underlying solid-state physics and synthesis of multiferroic materials have restored the tremendous potential of the field. For industrial application of multiferroic-based devices, the coupling of ferroelectric and ferromagnetic orders in multiferroic materials must be achieved at RT. So far, BiFeO3 is the only single-phase RT multiferroic material that is ferroelectric while displaying antiferromagnetic order. Nevertheless, BiFeO3 exhibits reasonable magnetoelectric coupling.19 It is an example of a direct multiferroic material, meaning application of a magnetic or electric field will directly alter the magnetization or electric polarization of the system, as opposed to the indirect effects observed in composite multiferroic materials.
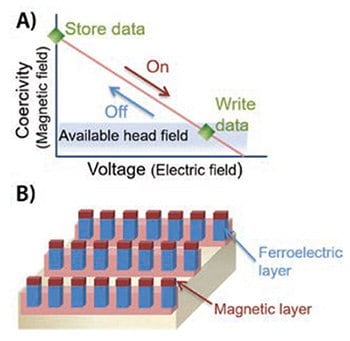
Figure 3. A) Working principle for electrically assisted magnetic recording and B) arrays of magnetic ferroelectric nanoislands with patterned bottom electrodes (pink) for purely electrically driven magnetization reversal in a selected storage unit.
In ABX3 perovskites like BiFeO3, lone electron pairs of the A cation distort the geometry of the BX3 octahedra. This “lone pair” effect is the key to its multiferroicity. It has been demonstrated that ferroelastic rather than ferroelectric domain switching plays a dominant role in multiferroic BiFeO3. But, control of the elastic interactions is a major challenge as it destabilizes the small switched volumes inducing back-switching at zero electric field and loss of non-volatile memory. To eliminate the backswitching, Eom et al. first reported the use of isolated monodomain BiFeO3 islands. Geometric frustration is another significant mechanism for multiferroics, primarily observed in YMnO3.20 Long-range dipole–dipole interactions and rotations of oxygen atoms facilitate a stable ferroelectric state in YMnO3. In this hexagonal manganite, the unexpected clamping of ferroelectric domain walls with the magnetic domain walls is driven by a unique lattice distortion, trimerization. Transmission electron microscopy and conductive atomic force microscopy characterization revealed remarkable “cloverleaf” defects where all six structural domains of YMnO3 merge. The electric polarization changes its sign six times along a loop enclosing these defect sites, creating an elegant demonstration of the geometric frustration mechanism. Another significant mechanism supporting multiferroicity is charge-ordering, particularly found in the RFe2O4 family of compounds (R = rare-earth element from Dy to Lu and Y). In LuFe2O4, the crystal structure contains alternate packing of triangular lattices of rare-earth elements, iron, and oxygen. Equal amounts of Fe2+ and Fe3+ occupy equivalent sites in the lattice. Consequently, RFe2O4 is a charge-frustrated system of triangular lattices because the pairing of opposite charges (Fe2+ and Fe3+) based on Coulomb’s law causes degeneracy in the lowest energy for charge configuration. These materials facilitate efficient electron transport and, hence, less coupling of polarization switching with lattice distortion. Therefore, the RFe2O4 class of multiferroics may be a key milestone to design fatigue-free charge capacitors. Multiferroic manganites, RMnO3 (R = rare-earth element) and TbMn2O4, on the other hand, exhibit spin-effect based magnetoelectricity. In these materials, magnetic long-range order and the lack of reflection symmetry in the arrangement of magnetic dipoles is responsible for ferroelectricity. The frustrated-spin systems show remarkable magnetocapacitance, opening new routes for spintronic applications.
In summary, multiferroic materials demonstrate a complex interplay between structural, electric, and magnetic properties, which is a key milestone toward multifunctional spintronic devices. Several promising multiferroic materials like Y-type hexaferrite [or (Ba, Sr)2Zn2Fe12O22, Ba2Mg2Fe12O22], Z-type hexaferrites (or Sr3Co2Fe24O41 and CuO), GdFeO3, CdCr2S4, and indium perovskites have been reported thus far. However, RT multiferroicity in single-phase materials has been challenging to achieve. The general focus in the field is shifting toward advanced characterization techniques, better thin-film fabrication tools, device architecture, and understanding of the domain-interface effects.
Perspective
Spintronics has far-reaching potential in both memory and logic computing devices because it exploits the phenomena that interlink the spin and charge degrees of freedom. Currently half-metallicity at RT and realizing perpendicular anisotropy in nanoscale devices at RT are the two key targets for the synthesis of new spintronic materials.21 With breakthroughs in the fundamental understanding of solid-state physics and fast-paced advancements in material development, these goals are soon to be met, as summarized in this review. Based on the current state of the research, we can anticipate all-Heusler and all-oxide junctions to be implemented in MRAM and high-frequency devices in the foreseeable future (Figure 4).
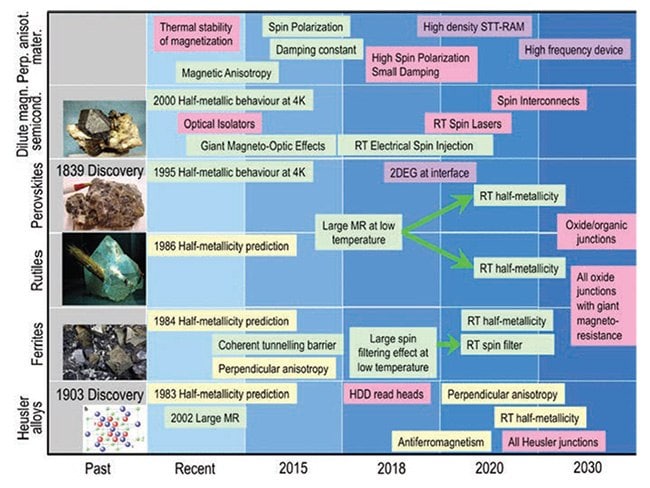
Figure 4.Roadmap for magnetic spintronic materials.3
Acknowledgments
This work was supported by NSF Grant no. ECCS-1509875. We gratefully acknowledge UA-MINT Center and Center for Integrated Nanotechnologies, Los Alamos National Laboratory for additional support. SP thanks CECS, UT Chattanooga for support.
Materials
References
如要继续阅读,请登录或创建帐户。
暂无帐户?