Magnetic Nanoparticles Synthesis
Riddhiman Medhi, Pannaree Srinoi, Tingting Liu, Hung-Vu Tran, Maria D. Marquez, T. Randall Lee
Department of Chemistry and the Texas Center for Superconductivity, University of Houston
Material Matters, 2020, 14.4
Read more about
- Introduction
- Iron Oxide Nanospheres
- Iron Oxide Nanocubes
- Applications in Magnetic Biosensing
- Conclusions
- Acknowledgements
- Related Products
- References
Introduction
Magnetic nanoparticles (MNPs) are widely used in biomedical applications such as biosensing, bioimaging, hyperthermia, and drug delivery.1 MNPs exhibit properties that are noticeably different from their bulk materials due to the particle size closely approaching the domain size as well as an increased surface-to-volume ratio afforded by the smaller dimensions.2 Given that biosensors are devices that provide both qualitative and quantitative information regarding the composition of the molecules in which the sensor is situated,3 biosensing applications require specific properties that strongly depend on size, shape, functionality, stability, and magnetization of the MNPs, as summarized in Figure 1.
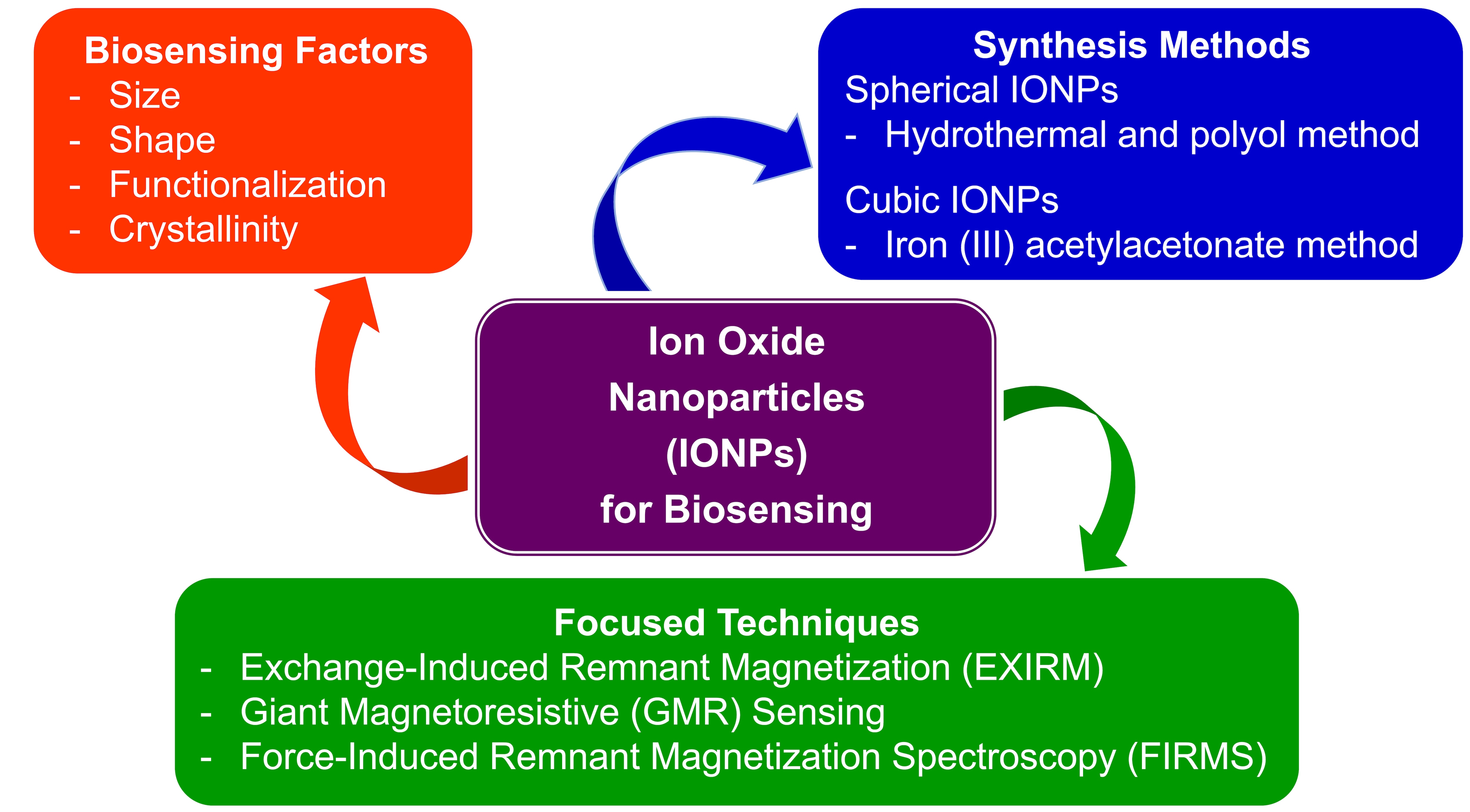
Figure 1.Magnetic nanoparticles for biosensing: techniques, factors affecting magnetization, and MNP synthetic methods.
Typically, MNPs are classified as diamagnetic, paramagnetic, ferromagnetic, ferrimagnetic, antiferromagnetic, and superparamagnetic based on intrinsic magnetic dipoles and the net magnetization in the presence and absence of an external magnetic field.2 In the presence of an external magnetic field, the maximum magnetization observed when the magnetic moment of the MNPs is aligned along the direction of the external magnetic field is defined as saturation magnetization (Ms).4 Upon removal of an external magnetic field, the magnetic moment of MNPs retains the previous direction and magnetization, which is defined as remnant magnetization (Mr). In general, MNPs with high Ms values are preferred for biosensing applications due to their high sensitivity, which provides enhanced sensing efficiency;5 furthermore, Ms values generally increase with the size of the MNPs. Even though there is no clear consensus with regard to shape, some reports have concluded that the greater biosensing capacity of cubic vs spherical MNPs arises from the greater crystallinity of the former.2
Several factors must be considered in the design of MNPs for specific biosensing applications. These include determining a suitable size and shape, modifying the MNPs with biocompatible ligands, optimizing the magnetic properties, and identifying suitable magnetic detection techniques to evaluate the effectiveness of the MNPs. Iron oxide nanoparticles (IONPs) are one of the most common metal oxide MNPs used in biosensing, due to their biocompatibility, low toxicity, strong superparamagnetism, good catalytic activity, and simple preparation processes.1,4 However, commercially available MNPs, particularly IONPs, are magnetically weak, which limits their sensitivity for magnetic detection. Consequently, the design and synthesis of IONPs with well controlled size and shape have been pursued in order to optimize their inherent magnetic and electrical properties in efforts to generate sensors with high sensitivity. The most common techniques used to synthesize IONPs are wet chemical methods that include co-precipitation, thermal decomposition, and hydrothermal methods.4 Various researchers are continuing to explore new methods for the synthesis of IONPs with controllable size and shape to study the effect of morphology on the magnetic properties in biosensing applications. In this article, we evaluate the synthetic procedures used to obtain homogeneous Fe3O4 nanospheres and nanocubes. We also discuss the effect of shape, size, and incorporation of a second metal (i.e., Fe3O4-based bimetallic MNPs) on the selectivity and sensitivity of IONP-based biosensors.
Iron Oxide Nanospheres
Various Fe3O4 nanostructures, such as nanospheres,6 nanocubes,6 nanorods,7 nanowires,8 and nanoplates9 have been reported and utilized in a wide variety of applications. For nonspherical Fe3O4 nanostructures, the shape is mainly controlled by the incorporation of surfactants, such as sodium oleate (O7501) and methyltrioctylammonium bromide (365718).9 For Fe3O4 nanospheres greater than 100 nm in diameter, the particles grow from a collection of nanocrystals that agglomerate into the final particle size.10 The hydrothermalpolyol method has proven to be highly effective for obtaining large IONPs (i.e., with diameters > 100 nm). Ethylene glycol (EG) (324558) and diethylene glycol (DEG) (32160) are two common polyols used to synthesize large, homogenous IONPs.6,11 Recently, Kolhatkar et al. reported the synthesis of a series of spherical IONPs ranging in diameter from 100 nm to 300 nm obtained by the hydrothermal-polyol method, using EG as the polyol and iron chloride hexahydrate (31232) as the source of iron.6 The authors varied reaction time, temperature, and stirring speed to prepare these IONPs. Similarly, Chen et al. reported a series of IONPs with an even wider size distribution — 120 nm, 440 nm, and 700 nm, shown in Figure 2.11 The authors indicated that for IONPs smaller than 200 nm, the reducing agent and solvent, in this case EG and DEG, were critical to the preparation of 120 nm IONPs. The authors also noted a higher number of agglomeration centers in the presence of DEG when the targeted size of the IONPs was larger than 200 nm; furthermore, EG provided a stronger reducing capability and better capacity for size control. Ultimately, the size of the IONPs can be affected by many factors, such as reaction temperature, pressure, time, concentration, stirring speed, reducing agent, and solvent.6,11
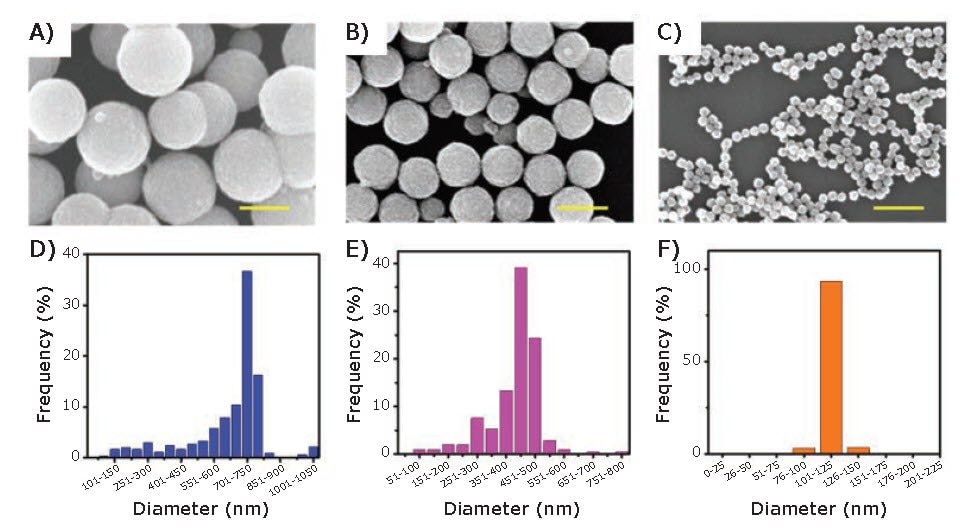
Figure 2.SEM images and size distributions of IONPs with diameters of 700, 440, and 120 nm, respectively. Scale bar: 500 nm.11
To prepare smaller (< 30 nm) spherical IONPs, co-precipitation, thermal decomposition, and hydrothermal methods are commonly used.10 Similar to the larger IONPs, the size of the particle can be controlled by adjusting the iron source, reaction temperature, as well as the pH of the solution.10 The co-precipitation method is considered to be one of the most effective methods to obtain IONPs smaller than 30 nm. Nevertheless, IONPs (< 30 nm) with excellent crystallite morphology can also be obtained by the thermal decomposition method as well as the hydrothermal method.10 Specifically, the thermal decomposition method requires an organic solvent with a high boiling point and a stabilizing agent, while the hydrothermal method requires the reaction to occur under high pressure (> 2000 psi) and high temperature (> 200 °C).10
Iron Oxide Nanocubes
There are two preferred routes for the synthesis of uniform iron oxide nanocubes (IONCs). One approach utilizes iron (III) acetylacetonate (517003) together with oleic acid (O1008) and benzyl ether (W237108). This approach was first used by Yang et al. in 2007,12 where mono-crystalline, magnetite-phase Fe3O4 nanocubes (with edge length of 15 nm) were synthesized using a mixture of Fe(acac)3, 1,2-hexadecanediol (213748), oleic acid, oleyl amine (O7805), and benzyl ether; the IONPs obtained from this method are shown in Figure 3A. Briefly, the above mixture was heated at 110 °C for 1 h under flowing Ar. The temperature was then raised to 200 °C and maintained at that temperature for 30 min. The reaction was further heated to a reflux temperature of ~290 °C at a heating rate 15 °C/min and refluxed for 1 h. The nanocubes were precipitated by adding ethanol (459836) and redispersed in a mixture of toluene (244511) and hexane (296090). This method offers at least two distinct advantages. First, it is a one-pot method, which is easier to execute than a seed-mediated growth method. Second, the size of the nanocubes can be adjusted simply by varying the heating parameters. For example, smaller nanocubes (e.g., 6.5 nm edge length) were obtained when the reflux temperature was quickly raised at a rate of 35 °C/min and a short reflux time of 20 min, due to the relatively faster rate of nucleation. Larger nanocubes (e.g., 30 nm edge length) were obtained via a slow rate of nucleation achieved by employing a 5°C/min heating rate and a longer reflux time of 3 h.
Kim et al. used a simplified version of this procedure to obtain even larger nanocubes with edge lengths of 79 nm, 110 nm, and 160 nm, as illustrated in Figures 3B and 3C.13 For the 79 nm cubes, a degassed mixture of Fe(acac)3, oleic acid, and benzyl ether was heated to 290 °C at 20 °C/min and then maintained at this temperature for 30 min. Toluene and hexane were added to this solution after cooling to precipitate the particles. Truncated cubes, with an edge length of 110 nm, were obtained by reducing the amount of benzyl ether; separately, by increasing the reaction time from 1.5 h to 2 h, cubes with an edge length of 160 nm were obtained.
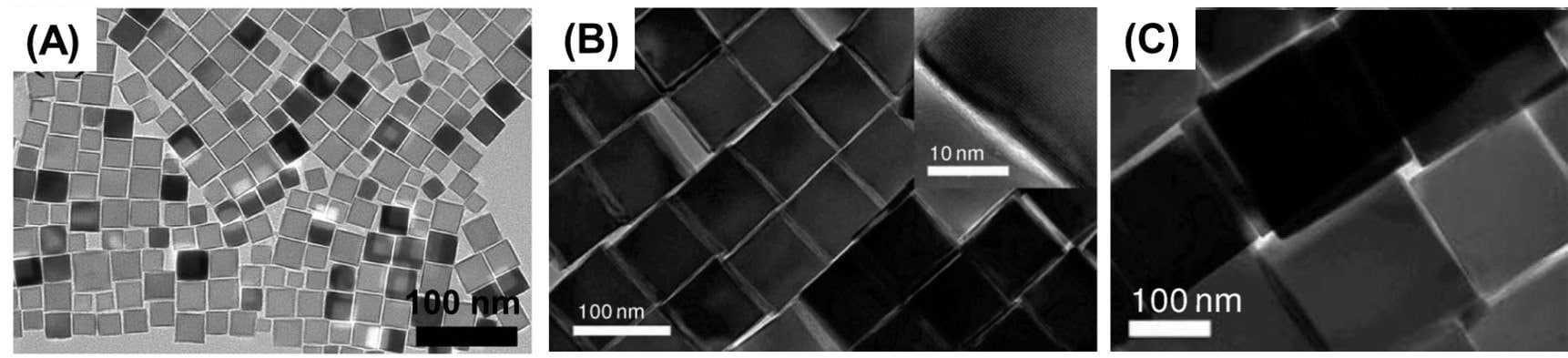
Figure 3.TEM images of IONCs synthesized using the ether-based reflux method by A) Yang et al. 12-13
Nanocubes are formed via fast growth along the [111] directions, and the projected surfaces of the nanocubes are (100) planes. Moreover, the versatile nature of this method for producing nanocubes of varying sizes—both small and large—was further illustrated by a slight modification of the recipe by adding 4-biphenylcarboxylic acid (B34729) along with a slightly greater amount of oleic acid to produce nanocubes with an edge length of 22 nm.13 Given its versatility, many researchers have made minor modifications to this method to obtain various IONCs.6,14 Kolhatkar et al. simplified this method further by using Fe(acac)3 and oleic acid heated in benzyl ether to 290 °C.6 By varying the reactant concentration and reaction time, these researchers were able to obtain even larger Fe3O4 nanocubes with body diagonals of 135, 150, 175, and 225 nm (edge lengths 80, 85, 100, and 130 nm, respectively), as shown in Figure 4. In a separate study, a reduced temperature profile in a magnesium acetate-assisted modification of this method also led to the successful synthesis of nanocubes. By adjusting the sodium oleate concentration, nanocubes with edge lengths of 22 nm, 36 nm, and 57 nm were obtained with a size deviation of less than 10%.15
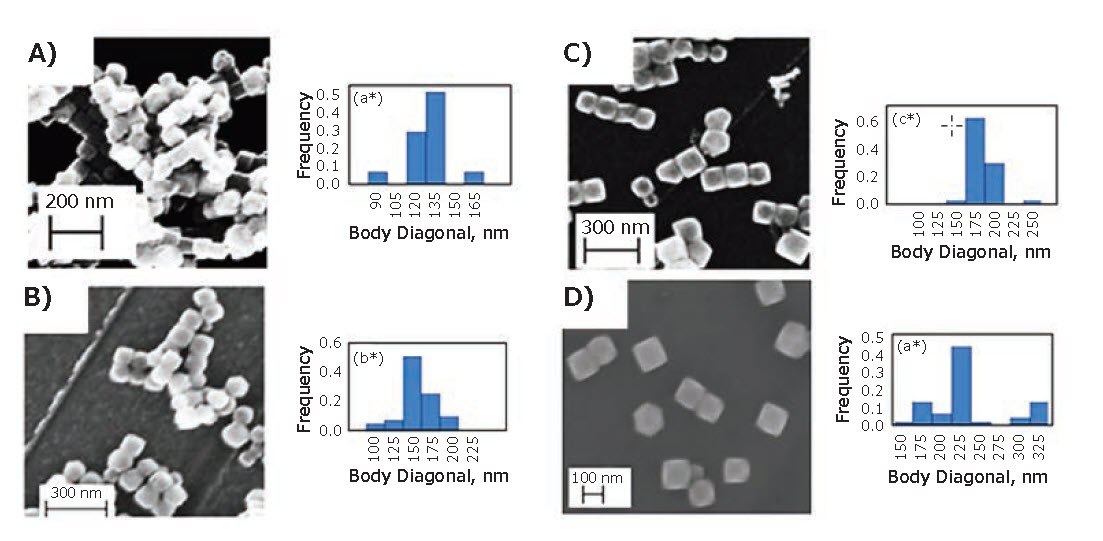
Figure 4.SEM images and respective size distribution plots for IONCs synthesized by Kolhatkar et al.6
Alternatively, IONCs with edge lengths of ~70 nm have also been synthesized using ferrocene as the iron source.16 In this method, ferrocene (F408) and polyvinylpyrrolidone (81420) were dissolved in water and alcohol followed by the slow addition of hydrogen peroxide. The mixture was then transferred to a Teflon-lined stainless-steel autoclave and placed in an oven at 230 °C for 24 h, and then ambiently cooled to room temperature. Notably, this method produces some polyhedron-shaped particles in addition to the cubes. Nevertheless, the ether-based reflux method remains as the preferred method for the synthesis of Fe3O4 nanocubes across multiple research groups due to its ease and reliability for producing cubic nanoparticles.6,14,15
Applications in Magnetic Biosensing
MNPs as biosensing tools rely on a variety of magnetic detection techniques, which can be classified into two classes: volumetricbased techniques and surface-based techniques. Volumetricbased sensors, such as the planar Hall effect (PHE) and nuclear magnetic resonance (NMR) systems, provide simple and rapid sample preparation and detection. On the other hand, surfacebased sensors, such as giant magnetoresistance (GMR) and tunnel magnetoresistance (TMR) sensors, offer extremely low detection limits but require laborious sample preparation. Furthermore, superconducting quantum interference devices (SQUIDs) and atomic magnetometers (AMs) are highly sensitive instruments that can be operated using either technique, depending on the targeted application.4 These biosensing methods rely on the magnetic properties of the material, which can be tuned by varying factors such as the size, shape, and composition of the particles.2 In the following sections, we focus our discussion on the effects of size, shape, and composition on the magnetic properties of MNPs and ultimately on the sensing ability of these nanomaterials.
The relationship between particle size and the performance of MNPs in biosensors was recently investigated by Chen et al.11 Specifically, Chen et al. synthesized magnetic iron oxide particles with diameters of 120, 440, and 700 nm, and then functionalized their surfaces with streptavidin for use in the specific and sensitive detection of proteins. Biofunctionalization of the MNPs was achieved via a silica-coating (from tetraethylorthosilicate, 333859), followed by the growth of a carboxy-terminated polymer generated from 3-(trimethoxysilyl) propyl methacrylate (440159) followed by carbodiimide (03449) coupling to the pendant amino groups on streptavidin.
The magnetic signals and responses improved significantly as the particle size was increased and were greater than those of commercially available Dynabeads. As a proof of concept, these functionalized-magnetic particles were tested in the specific detection of a targeted protein (IgG2a) in solution by displacing a magnetically labeled protein (IgG1) on the receptor, where the driving force of the exchange arose from the difference in the binding affinity of the protein to the surface-immobilized receptor (i.e., the binding affinity of IgG2a was greater than that of IgG1). These highly sensitive and specific label-free protein detection experiments were performed by using the exchange-induced remnant magnetization (EXIRM) method, which is derived from force-induced remnant magnetization spectroscopy (FIRMS).17 The synthesized MNPs showed sensitivity improvements of 9-, 4-, and 2-fold for the 700, 400, and 100 nm magnetic particles, respectively, when compared to commercially available Dynabeads.
Kolhatkar et al. also investigated the relationship between the morphology of IONPs and their magnetic sensing ability.6 Specifically, Fe3O4 nanocubes and nanospheres of tunable size were synthesized to obtain particles with either equivalent volumes or dimensions (i.e., body diagonal/diameter). The magnetic properties of these MNPs were compared by using vibrating sample magnetometry (VSM), which revealed a Ms and coercivity for the nanocubes 1.4–3 and 1.1–8.4 times higher than those of the nanospheres, on a same-volume and same-body diagonal/diameter basis, respectively. Analysis by X-ray diffraction (XRD) and transmission electron microscopy (TEM) showed that the nanocubes were more crystalline than the nanospheres; the nanospheres are polycrystalline while the nanocubes grow as largely monocrystalline (Figure 5A). Consequently, the higher crystallinity of the nanocubes is responsible for the higher Ms and higher Curie temperature when compared to the corresponding nanospheres. Furthermore, the sensing potential of the synthesized MNPs were evaluated via GMR sensing and FIRMS. Both techniques revealed a distinct sensitivity advantage for the nanocubes when compared to the nanospheres. This result was attributed to the higher magnetization and binding affinity of the nanocubes (Figure 5B).
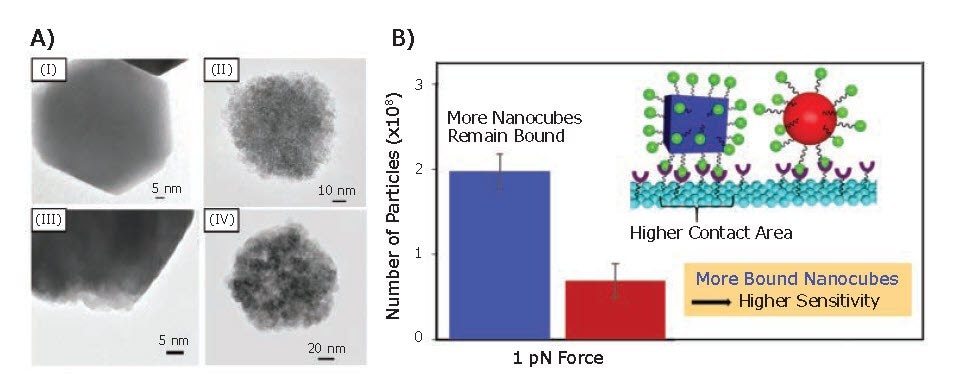
Figure 5.Magnetic sensing ability of Fe3O4 nanocubes and nanospheres. A) TEM images of (I) 150 nm cubic Fe3O4 MNPs, (II) 100 nm spherical Fe3O4 MNPs, (III) 135 nm cubic Fe3O4 MNPs, and (IV) 135 nm spherical Fe3O4 MNPs. B) Number of particles on the sensor at 1 pN and contact area for cubic MNPs (blue) compared to spherical MNPs (red).6
In addition to single-component MNPs, magnetic bimetallic nanoparticles have also been used as biosensing materials.18 These nanoparticles typically exhibit higher magnetic moments than their single-component counterparts. Consequently, bimetallic nanoparticles usually offer improved sensitivities than the single-component MNPs. However, similarly to singlecomponent IONPs, modification of the particle composition and structure influences the magnetic response. For example, Kolhatkar et al. synthesized and studied cubic silica-coated FeCo MNPs and found that as the size of the particles was systematically increased, the magnetic signal and response correspondingly increased.19
Conclusions
To produce MNP-based biosensors with high sensitivity, the key factor is the availability of MNPs with strong magnetic responses. As demonstrated herein, sensor responses can be enhanced simply by adjusting the size and morphology of the MNPs, where larger particles outperform smaller particles, and particles with high crystallinity (e.g., nanocubes) outperform those with low crystallinity (e.g., nanospheres). Various synthetic methods have been developed to obtain both large (> 100 nm) and small (< 30 nm) MNPs having either spherical or cubic shape with narrow size distributions and large crystallite sizes. Moreover, the further development of new MNPs can lead to novel approaches to MNP-based sensing, such as the recently demonstrated “lossof-signal” approach where IONPs are converted, in situ, to a non-magnetic product.20
Acknowledgments
This research was generously supported by the Robert A. Welch Foundation (Grant No. E-1320), the Air Force Office of Scientific Research (AFOSR FA9550-18-1-0094), and the Texas Center for Superconductivity at the University of Houston.
References
如要继续阅读,请登录或创建帐户。
暂无帐户?